Humankind caught its first glimpse of a black hole on April 10, 2019. The Event Horizon Telescope (EHT) team, which uses an Earth- spanning network of radio observatories acting in concert, shared images it had captured of an apparent black hole with 6.5 billion times the mass of our sun in the center of the nearby M87 galaxy. This was a breathtaking achievement—our first view of one of the most mysterious objects in the universe, long predicted but never directly “seen.” Even more exciting, the images, and the observations that should follow, are beginning to provide new clues about one of the deepest puzzles in physics.
This enigma is the “paradox” of what happens to information in a black hole. By investigating this question, physicists have discovered that the mere existence of black holes is inconsistent with the quantum-mechanical laws that so far describe everything else in our universe. Resolving this inconsistency may require a conceptual revolution as profound as the overthrow of classical physics by quantum mechanics.
Theorists have explored many ideas, but there has been little direct evidence to help resolve this problem. The first image of a black hole, however, begins to offer actual data to inform our theories. Future EHT observations—especially those that can show how black holes evolve over time—and recent detections of colliding black holes by gravitational-wave observatories could provide important insights and help to usher in a whole new era of physics.
On supporting science journalism
If you're enjoying this article, consider supporting our award-winning journalism by subscribing. By purchasing a subscription you are helping to ensure the future of impactful stories about the discoveries and ideas shaping our world today.
The information Problem
Though deeply mysterious, black holes seem to be ubiquitous in the cosmos. The EHT observations and the gravitational-wave measurements are just the latest and most robust evidence that black holes, despite sounding fantastical, do indeed appear to be real—and remarkably common. Yet their very existence threatens the present foundations of physics. The basic principles of quantum mechanics are thought to govern all the other laws of nature, but when they are applied to black holes they lead to a contradiction, exposing a flaw in the current form of these laws.
The problem arises from one of the simplest questions we can ask about black holes: What happens to stuff that falls into them? We need a little refinement here to fully explain. First, according to our present quantum-mechanical laws, matter and energy can shift between different forms: particles can, for example, change into different kinds of particles. But the one thing that is sacred and never destroyed is quantum information. If we know the complete quantum description of a system, we should always be able to exactly determine its earlier or later quantum description with no loss of information. So a more precise question is, What happens to quantum information that falls into a black hole?
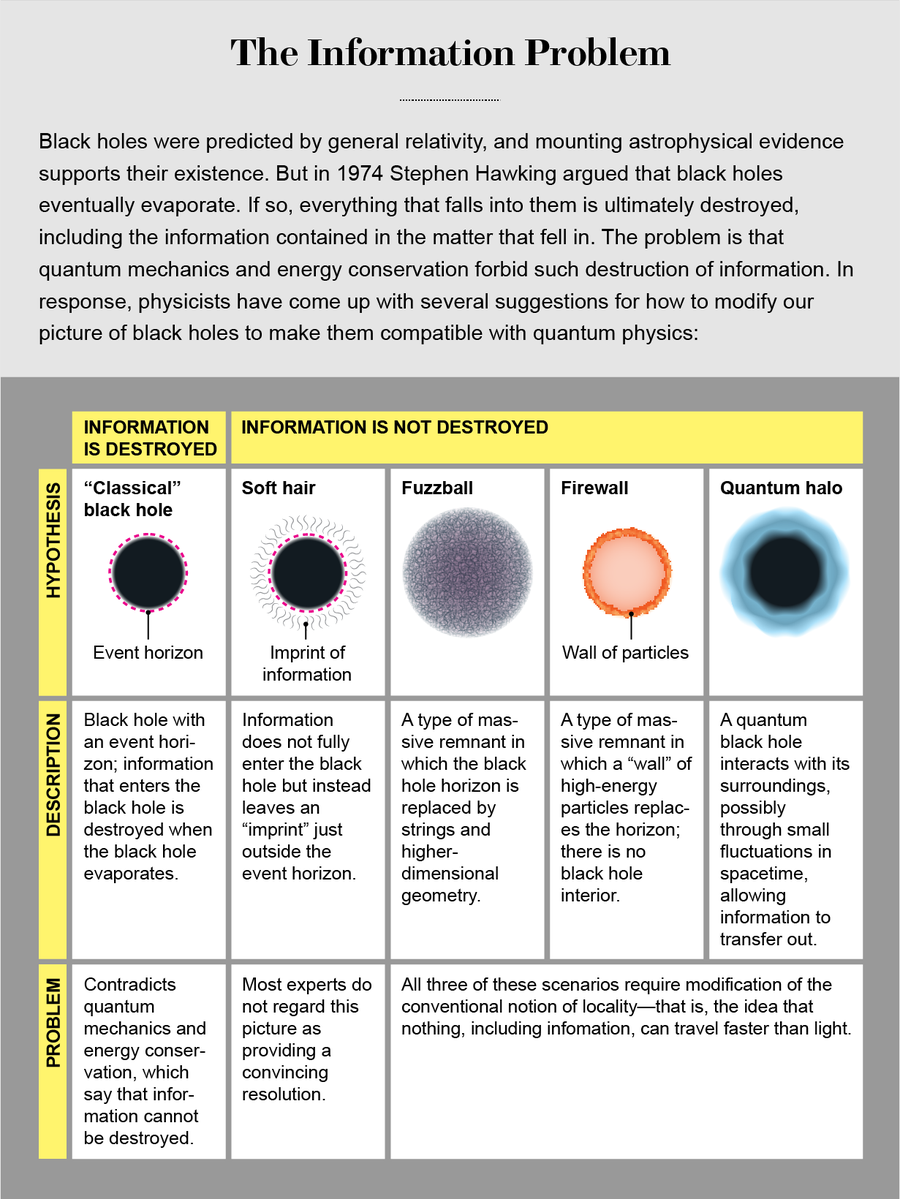
Credit: Amanda Montañez
Our understanding of black holes comes from Albert Einstein’s general theory of relativity, which describes gravity as arising from the curvature of space and time; a common visualization of this idea is a heavy ball deforming the surface of a trampoline. This warping of spacetime causes the trajectories of massive bodies and light to bend, and we call that gravity. If mass is sufficiently concentrated in a small-enough vicinity, the nearby spacetime deformation is so strong that light itself cannot escape a region inside what we call the event horizon: we have a black hole. And if nothing can travel faster than light—including information—everything must get stuck inside this boundary. Black holes become cosmic sinkholes trapping information along with light and matter.
But the story becomes stranger. What may be Stephen Hawking’s greatest discovery is his 1974 prediction that black holes evaporate. This finding also led to the startling idea that black holes destroy quantum information. According to quantum mechanics, pairs of “virtual particles” pop into existence all the time, everywhere. Typically such a pair, consisting of a particle and its antimatter counterpart, quickly annihilates itself, but if it forms near the horizon of a black hole, one particle might pop up inside this boundary and the other outside. The outside particle can escape, carrying away energy. The law of energy conservation tells us that the black hole has thus lost energy, so the emission of such particles causes the black hole to shrink over time until it completely disappears. The problem is that the escaping particles, known as Hawking radiation, carry essentially no information about what went into the black hole. Therefore, Hawking’s calculations appear to show that quantum information that falls into a black hole is ultimately destroyed—contradicting quantum mechanics.
This revelation initiated a deep crisis in physics. Great advances have followed from previous such crises. For instance, at the beginning of the 20th century, classical physics seemed to predict the inevitable instability of atoms, in obvious contradiction to the existence of stable matter. That problem played a key role in the quantum revolution. Classical physics implied that because orbiting electrons within atoms are constantly changing direction, they continually emit light, causing them to lose energy and spiral into the nucleus. But in 1913 Niels Bohr proposed that electrons actually travel only within quantized orbits and cannot spiral in. This radical idea helped to establish the basis of quantum mechanics, which fundamentally rewrote the laws of nature. Increasingly it seems that the black hole crisis will similarly lead to another paradigm shift in physics.
Quantum Alternatives
When Hawking first predicted black hole evaporation, he suggested that quantum mechanics must be wrong and that information destruction is allowed. Yet physicists soon realized this change would require a drastic breakdown of the law of energy conservation, which would disastrously invalidate our present description of the universe. Apparently the resolution must be sought elsewhere.
Another early idea was that black holes do not completely evaporate but instead stop shrinking at a tiny size, leaving behind microscopic remnants containing the original information. But, scientists realized, if this were true, basic properties of quantum physics would predict catastrophic instabilities causing ordinary matter to explode into such remnants, also contradicting everyday experience.
Obviously something is very wrong. It is tempting to conclude that the flaw is in Hawking’s original analysis and that somehow information does escape a black hole emitting Hawking radiation. The challenge here is that this scenario would conflict with a foundational concept of present-day physics, the principle of locality, which states that information cannot move from one place to another superluminally—that is, faster than the speed of light. But according to our definition of black holes, the only way to escape one is to travel faster than light, so if information does escape, it must be doing so superluminally, in conflict with locality. In the four decades since Hawking’s discovery, physicists have tried to find a loophole to this argument that stays within conventional physics, but none has emerged.
The closest attempt was a 2016 proposal by Hawking, Malcolm Perry and Andrew Strominger, who suggested that a mistake in the original analysis implies information never fully enters a black hole and instead leaves a kind of imprint in the form of what they called “soft hair” outside it. Closer examination seems to be closing this loophole, however, and most experts do not believe this can be the answer. In short, more radical steps appear to be needed.
An obvious idea is that there is some unknown physics that prevents true black holes from existing at all. The conventional picture of black hole formation says that when very large stars burn out and die, their mass collapses under the force of gravity into a black hole. But what if they never reach that stage and actually transform into objects with “better” behavior? In fact, we know that when lower-mass stars such as our sun burn out and collapse, they do not form black holes and instead form dense remnants—for example, white dwarfs or neutron stars. Perhaps some unknown laws of physics also prevent larger stars from forming black holes and instead lead them to become a kind of “massive remnant”—something more like a neutron star than a black hole.
The problem with this suggestion is that we cannot explain what would stabilize such objects—no known physics should prevent their continued collapse under gravity, and any imagined physics that did would apparently require superluminal signaling from one side of the collapsing matter to the other. In fact, conventional large black holes can form from very low-density matter. To illustrate, if the 6.5-billion-solar-mass black hole in M87 arose from the collapse of a dust cloud (which is theoretically possible, although the actual process was apparently more complex), it would have happened when the dust reached the density of air at the top of Mount Everest. (Air on top of Everest does not form a black hole because there is not enough of it; one would require an accumulated 6.5 billion solar masses.) Some drastic and superluminal new physical process would need to take over in such a low-density regime to instantly convert the collapsing cloud into a massive remnant instead of allowing a black hole to form.
A related idea is that something could cause black holes to change into massive remnants containing the original information after they form but long before they evaporate. But once again, this story requires nonlocal transfer of information from the interior of the initial black hole to the final remnant.
Despite their problems, physicists have explored versions of both these scenarios. For example, in 2003 Samir Mathur put forward a proposal based on string theory, which posits that fundamental particles are tiny strings. His idea is that a black hole transforms into a “fuzzball,” a kind of massive remnant, or that a fuzzball forms instead of a black hole in the first place. Thanks to the complicated physics of string theory and its allowance for more than the traditional four dimensions of spacetime, fuzzballs might have a complex higher-dimensional geometry; instead of the sharp traditional boundary of a black hole at the event horizon, a fuzzball would have a fuzzier and larger boundary where one encounters strings and higher-dimensional geometry.
Alternatively a more recent version of a remnant scenario is the proposal that instead of a black hole with an event horizon, a massive remnant forms with a surface “firewall” of high-energy particles where the horizon would be. This firewall would incinerate anything that encountered it, turning it into pure energy that added to the firewall. Both the firewall and the fuzzball, though, share the problem of needing locality violation, and the resulting objects would have other properties that are very hard to explain.
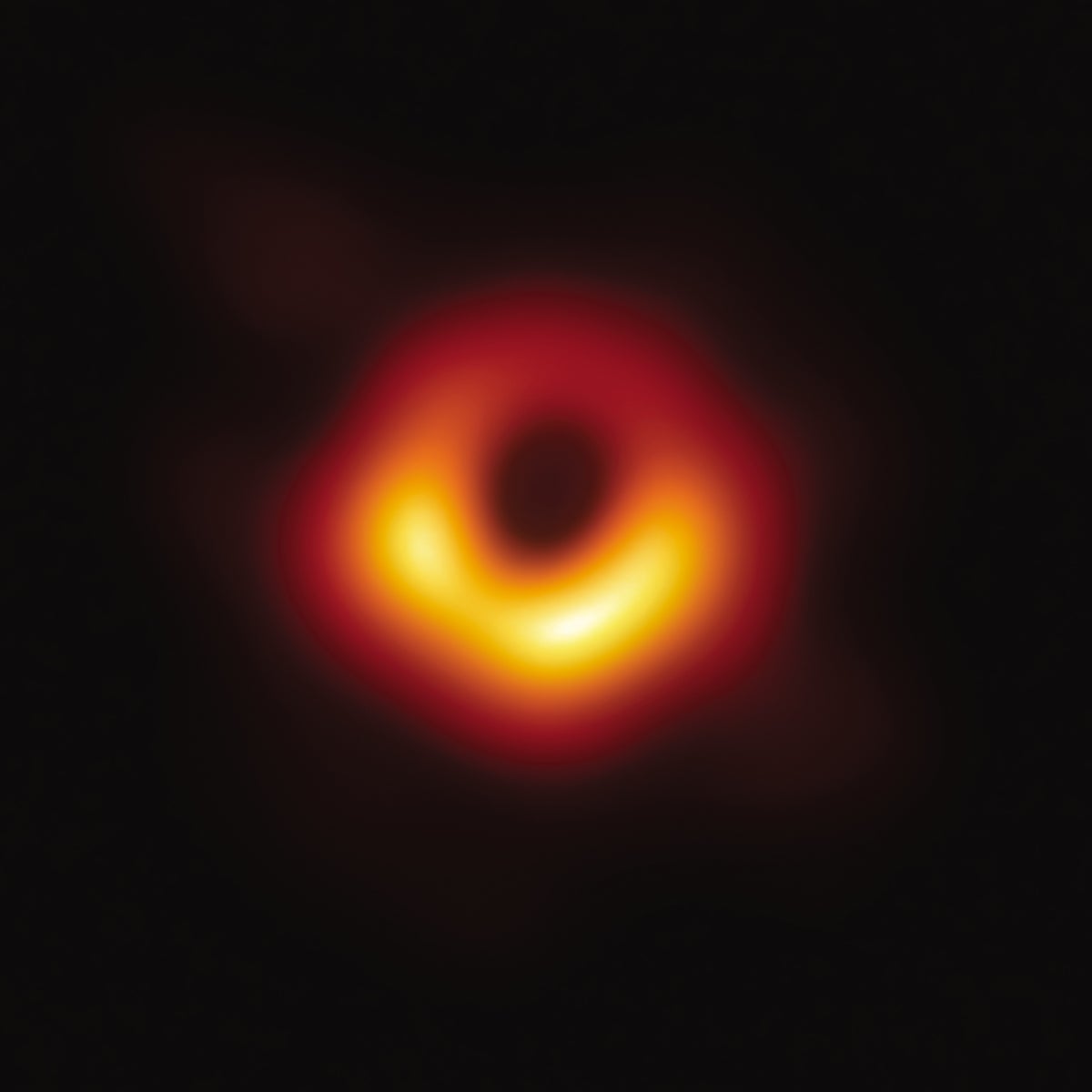
Gravity bends light around an apparent black hole at the center of the M87 galaxy in this seminal image from the Event Horizon Telescope.
Credit: Event Horizon Telescope [CC BY 4.0 (https://creativecommons.org/licenses/by/4.0)]
Modifying Locality
A common thread in massive-remnant proposals is that saving quantum mechanics appears to require violation of the locality principle. But doing so carelessly is expected to be as disastrous as modifying quantum mechanics and, in fact, typically leads to another paradox. Specifically, the laws of relativity say that if you send a faster-than-light signal in empty, flat space, observers traveling past you at a high-enough speed will see the signal going backward in time. The paradox arises because this superluminal signaling then allows you to send a message into your past, for example, asking someone to kill your grandmother before your mother is born.
Even though this kind of answer appears to contradict fundamental physical principles, it is worth a closer look. Modifying locality seems crazy, but we have not found an alternative that does not. The severe nature of the black hole crisis strongly suggests a resolution via some subtle violation of the locality principle, one that does not produce such paradoxes. Put differently, quantum mechanics implies information is never destroyed, so information that falls into a black hole must ultimately escape, possibly through some new, subtle “delocalization” of information that might become clear when we can finally find a way to unify quantum mechanics and gravity—one of the most profound problems of present-day physics. In fact, we have other reasons to think such a subtlety could be present. The very idea of localized information—that it can exist in one place and not in another—is more delicate in theories that include gravity than in those that do not, because gravitational fields extend to infinity, complicating the concept of localization.
If information does escape black holes, it might not require a change as obvious and abrupt as the formation of a massive remnant, whether fuzzball, firewall or another variant. The growing evidence for black holes suggests there are objects in the universe that look and act a lot like classical black holes, without large departures from Einstein’s predictions. Is Einstein’s general relativity so drastically wrong in its description of black holes, or might there be some more innocuous, currently unknown effects that delocalize information and allow it to leak from black holes, avoiding such a dramatic failure of the entire spacetime picture?
In my theoretical work, I have found two versions of such effects. In one, the geometry of spacetime near a black hole is altered, making it bend and ripple in a way that depends on the information in the black hole—but gently, so that it does not, for example, destroy an astronaut falling through the region where the horizon would ordinarily be found. In this “strong, nonviolent” scenario, such shimmering of spacetime can transfer the information out. Interestingly, I have also found that there is a subtler, intrinsically quantum way for information to escape the black hole. In this “weak, nonviolent” scenario, even tiny quantum fluctuations of the spacetime geometry near the black hole can transfer information to particles emanating from the hole. The fact that the information transfer is still large enough to save quantum mechanics is related to the huge amount of possible information a black hole can contain. In either picture, a black hole effectively has a “quantum halo” surrounding it, where interactions pass information back to its surroundings.
Notably, these scenarios, despite appearing to require superluminal travel of information, do not necessarily produce a grandmother paradox. The information signaling here is tied to the existence of the black hole, which has a spacetime geometry that is different from that of flat space, so that the earlier argument about communicating with the past no longer holds. These possibilities are tantalizing from another perspective: the locality principle is also what prohibits our own faster-than-light travel; the quantum mechanics of black holes seems to be telling us there is something wrong with the present formulation of this principle.
Rewriting the Laws of Physics
So far such a quantum-halo scenario has not been predicted by a more complete theory of physics that reconciles quantum mechanics with gravity, but it is strongly indicated by the need to resolve the problem and by assumptions based on what we see. If such a scenario is correct, it probably represents an approximate description of a deeper reality. Our very notions of space and time, which underlie the rest of science, appear to require significant revision. The present work to understand black holes may be akin to the first attempts to model the physics of the atom by Bohr and others. Those early atomic descriptions were also approximate and only later led to the profound theoretical structure of quantum mechanics. Although modifying locality seems impossible, we might find solace by noting that the laws of quantum mechanics also seemed quite impossible to the classical physicists grappling with their discovery.
Given the immense challenge in sorting out the story of quantum black holes and the more complete theory describing them, physicists are eager for experimental and observational evidence to help guide us. The exciting recent advances have given humankind two direct observational windows on black hole behavior. In addition to the EHT’s images of black holes, the Laser Interferometer Gravitational-wave Observatory (LIGO) and its companion facilities have begun to detect gravitational waves from collisions between apparent black holes. These waves carry valuable information with them about the properties and behavior of the objects that created them.
From a naive viewpoint, it seems preposterous that the EHT or LIGO could detect any departure from Einstein’s description of black holes. Traditionally his theory has been expected to need modification only when spacetime curvatures become extremely large, near the center of a black hole; in contrast, curvatures are very weak near the horizon of a large black hole. But the information crisis I have described suggests otherwise. A large part of the theoretical community has now reached the consensus that some changes to the current laws of physics are needed to describe phenomena not just deep inside a black hole but all the way out past the horizon. We appear to have crossed the Rubicon. For the case of the black hole in M87, the distance at which we expect to find deviations from classical predictions is several times the size of our solar system.
Already LIGO and the EHT have ruled out wilder possibilities that could be considered in an attempt to give a logically consistent description of black holes. Specifically, if black holes were replaced by massive remnants more than about twice the diameter of the supposed black hole, we would have seen signs in the data from both experiments. In the case of the EHT, much of the light that produced the now famous image comes from a region around one and a half times the diameter of the event horizon. And for LIGO, part of the gravitational-wave signal that we detect is likewise produced from the region where the colliding objects reach similarly small separations. Although study of these signals is still in early phases, the EHT and LIGO have revealed very dark and very compact objects that produce signals just like those predicted for unmodified black holes.
Still, it is important to investigate these signals more closely. Sufficiently careful analysis might in fact uncover more clues about the quantum physics of black holes. Even if no new effects are observed, we then have information that constrains possible descriptions of their quantum behavior.
Sufficiently large-diameter remnants are now ruled out, but what about remnant scenarios that modify the black hole description only very near the horizon? Although a complete discussion would require a fuller theory of these remnants—such as fuzzballs or firewalls—we have some initial indicators. Specifically, if these objects had radii barely larger than the radius of the corresponding black hole horizon, then it is likely that neither EHT nor LIGO observations would be able to reveal such a structure because very little light or gravitational radiation escapes from the region very near the horizon.
One possible exception is the possibility of gravitational “echoes.” As first suggested in 2016 by Vitor Cardoso of the University of Lisbon, Edgardo Franzin of the International School for Advanced Studies in Italy and Paolo Pani of Sapienza University of Rome, if two such remnants combine to form a final remnant that has similar properties, gravitational waves can reflect off the merged remnant’s surface and might be observed. Whereas most near-horizon scenarios are hard to rule out through observation, however, it is difficult to explain how such structures could be stable, instead of collapsing under their own weight to form black holes. Of course, this is a general problem for all massive-remnant scenarios, but it becomes even more challenging in the presence of the extreme forces in such a collision.
Prospects are better for testing some of the scenarios where new interactions behave like subtle modifications of spacetime geometry but extend well outside the horizon. For example, in the strong, nonviolent scenario, the rippling of a black hole’s quantum halo can distort light passing near the black hole. If this scenario is correct, the shimmering could cause distortions of the EHT’s images that change with time.
In my work with EHT scientist Dimitrios Psaltis, we found these changes could happen over roughly an hour for the black hole in the center of our galaxy. Because the EHT combines multihour observations into an average, such effects may be hard to see. But the relevant fluctuation time for the black hole in M87, which is more than 1,000 times larger, is more like tens of days. This work suggests we should look for these distortions by using longer-duration EHT observations than the project’s initial seven-day span. If the experiment found such distortions, they would be a spectacular clue to the quantum physics of black holes. If they do not appear, that will begin to point to the subtler weak quantum scenario or to something even more exotic.
The weak, nonviolent scenario is harder to test because of the relative smallness of the expected changes to the geometry. Yet preliminary investigation shows that this scenario can alter how gravitational waves are absorbed or reflected, possibly yielding an observable modification to gravitational-wave signals.
If either scenario is correct, we will learn more not only about what quantum black holes are but also about the deeper laws of nature. Right now we do not fully understand how to think about information localization when gravitational fields are present. Quantum physics suggests that spacetime itself is not a fundamental part of physics but instead arises only as an approximation of a more basic mathematical structure. Evidence for quantum black hole effects could help make this concept more concrete.
To learn more, it is important to extend and improve both EHT and gravitational-wave measurements. For the EHT, it would be useful to have significantly longer-duration observations, as well as images of other targets such as our galaxy’s central black hole, both of which are anticipated. For gravitational waves, more observations with increased sensitivity would be helpful and will be assisted when additional detectors come online in Japan and India, adding to the existing facilities in the U.S. and Europe. Furthermore, a strong complementary theoretical effort is needed to refine scenarios, to better clarify their origins and explanations, and to assess more thoroughly the question of how significantly they can affect EHT or gravitational-wave signals.
Whatever the resolution to the crisis, black holes contain crucial clues to the basic quantum physics of gravity, as well as to the very nature of space and time. Just as with the atom and quantum mechanics, a better understanding of black holes is likely to help guide the next conceptual revolution in physics. EHT and gravitational-wave observations have the potential to provide us with key information, either by ruling out quantum black hole scenarios or by discovering new phenomena associated with them.