Nordic Blue Carbon Ecosystems: Status and Outlook
- 1Department of Ecoscience, Aarhus University, Silkeborg, Denmark
- 2Arctic Research Centre, Aarhus University, Århus, Denmark
- 3Norwegian Institute for Water Research (NIVA), Oslo, Norway
- 4Department of Ecology, Environment and Plant Sciences, Stockholm University, Stockholm, Sweden
- 5School of Natural Sciences, Technology and Environmental Studies, Södertörn University, Huddinge, Sweden
- 6Department of Biological and Environmental Sciences, University of Gothenburg, Fiskebäckskil, Sweden
- 7Environmental and Marine Biology, Åbo Akademi University, Åbo, Finland
- 8Department of Biology, University of Southern Denmark, Odense M, Denmark
- 9Department of Science and Environment, Roskilde University, Roskilde, Denmark
- 10Flødevigen Research Station, Institute of Marine Research, His, Norway
- 11Department of Environmental Science - Atmospheric Emissions, Aarhus University, Roskilde, Denmark
- 12Conservation International, Arlington, VA, United States
- 13Icelandic Institute of Natural History, Borgum við Norðurslóð, Akureyri, Iceland
- 14TARI - Faroe Seaweed, Tórshavn, Faroe Islands
- 15Fiskaaling, Hvalvík, Faroe Islands
Vegetated coastal and marine habitats in the Nordic region include salt marshes, eelgrass meadows and, in particular, brown macroalgae (kelp forests and rockweed beds). Such habitats contribute to storage of organic carbon (Blue Carbon – BC) and support coastal protection, biodiversity and water quality. Protection and restoration of these habitats therefore have the potential to deliver climate change mitigation and co-benefits. Here we present the existing knowledge on Nordic BC habitats in terms of habitat area, C-stocks and sequestration rates, co-benefits, policies and management status to inspire a coherent Nordic BC roadmap. The area extent of BC habitats in the region is incompletely assessed, but available information sums up to 1,440 km2 salt marshes, 1,861 (potentially 2,735) km2 seagrass meadows, and 16,532 km2 (potentially 130,735 km2, including coarse Greenland estimates) brown macroalgae, yielding a total of 19,833 (potentially 134,910) km2. Saltmarshes and seagrass meadows have experienced major declines over the past century, while macroalgal trends are more diverse. Based on limited salt marsh data, sediment C-stocks average 3,311 g Corg m-2 (top 40-100 cm) and sequestration rates average 142 g Corg m-2 yr-1. Eelgrass C-stocks average 2,414 g Corg m-2 (top 25 cm) and initial data for sequestration rates range 5-33 g Corg m-2, quantified for one Greenland site and one short term restoration. For Nordic brown macroalgae, peer-reviewed estimates of sediment C-stock and sequestration are lacking. Overall, the review reveals substantial Nordic BC-stocks, but highlights that evidence is still insufficient to provide a robust estimate of all Nordic BC-stocks and sequestration rates. Needed are better quantification of habitat area, C-stocks and fluxes, particularly for macroalgae, as well as identification of target areas for BC management. The review also points to directives and regulations protecting Nordic marine vegetation, and local restoration initiatives with potential to increase C-sequestration but underlines that increased coordination at national and Nordic scales and across sectors is needed. We propose a Nordic BC roadmap for science and management to maximize the potential of BC habitats to mitigate climate change and support coastal protection, biodiversity and additional ecosystem functions.
Introduction
Vegetated coastal ecosystems including seagrass meadows, salt marshes, mangroves, and macroalgae are increasingly acknowledged for their contribution to sequestration and long-term storage of organic carbon (blue carbon, BC) and are, therefore, termed blue carbon habitats and ecosystems (Nellemann et al., 2009; Mcleod et al., 2011; Duarte et al., 2013, Krause-Jensen et al., 2018). Their capacity for long-term C-storage has made the management of these ecosystems relevant in relation to climate change mitigation; in addition, they constitute natural coastal protection by dampening wave energy and stabilizing and accreting sediments, thereby also contributing to climate change adaptation (Duarte et al., 2013; Macreadie et al., 2021). They also support biodiversity, including commercially important species, such as cod (Orth et al., 2020), and constitute coastal nutrient filters and sediment traps, which promote water quality and clarity (Aoki et al., 2020). These multiple functional roles and ecosystem services highlight the ecological and societal value of BC habitats (Barbier et al., 2011; Duarte et al., 2013; Smale et al., 2013; Gundersen et al., 2016). However, their location in the coastal zone where human pressures, such as eutrophication, fisheries and construction interact with climate change impacts such as heat waves and ocean acidification, render BC habitats among the most threatened on the globe (Orth et al., 2006; Waycott et al., 2009; Valiela et al., 2018; Dunic et al., 2021). Decline of the habitats due to diverse stressors put the C-reservoir at risk of erosion and to emit carbon dioxide (CO2), and thereby turn the C-sinks into sources (Lovelock et al., 2017; Salinas et al., 2020; Moksnes et al., 2021).
Because of the multiple functions of vital BC ecosystems, actions to protect and restore these environments present win-win-win scenarios for buffering climate change, protecting biodiversity, and mitigating eutrophication, and for supporting human wellbeing (food security etc.) (Duarte et al., 2013; Gattuso et al., 2018; Hoegh-Guldberg et al., 2019; Macreadie et al., 2021). Such BC strategies are therefore natural climate solutions in parallel to forest protection and reforestation on land (Macreadie et al., 2021), which can supplement direct emission reductions. Protection of existing BC habitats preserves both the C-sequestration capacity and the sediment BC stocks, while restoration of lost BC habitats can regenerate the C-sequestration capacity and, over the long-term, rebuild C-stocks (e.g. Orth et al., 2020). As restoration can be challenging and costly, especially for seagrasses (van Katwijk et al., 2016), prevention of loss has key management priority. At the global scale, the climate change mitigation potential of protection and restoration of mangroves, salt marshes and seagrass meadows could potentially represent 3% of current global emissions (Macreadie et al., 2021).
The C-sequestration capacity of salt marshes, seagrass meadows and mangroves exceeds that of green (terrestrial) forest ecosystems because of the combination of high ecosystem productivity, high potential for sedimentation of organic matter originating from the ecosystem itself (autochthonous) and from the surroundings (allochthonous), and the refractory nature of part of the organic matter and water-logged, anaerobic conditions limiting decomposition (Mcleod et al., 2011). The contribution of macroalgae to C-sequestration is more intricate to quantify than for seagrasses, salt marshes and mangroves. Unlike those systems that store C in sediments directly below them, macroalgal communities dominate rocky seafloor and their contribution to C-sequestration largely occurs through export to C-sinks beyond the habitat in fjord- and shelf sediments, and in the deep ocean, which is challenging to quantify (Krause-Jensen and Duarte, 2016; Pedersen et al., 2020). Management of macroalgal BC should consequently address both the macroalgal habitats and the BC sinks beyond the habitats (Krause-Jensen et al., 2018; Legge et al., 2020; Diesing et al., 2021).
The merit of protection and restoration of vegetated coastal ecosystems for climate action with co-benefits for biodiversity and human wellbeing underlines the importance of coordinated BC-strategies across sectors and regions. The Nordic region has a tradition of collaboration, e.g. under the auspices of the Nordic Council of Ministers, which is the official body for intergovernmental cooperation in the Nordic Region that seeks Nordic solutions wherever and whenever the countries can achieve more together than by working on their own. Such collaboration is also relevant regarding Nordic blue carbon ecosystems where joint efforts in science and management could benefit the region, and initiatives are already initiated. The study of vegetated coastal ecosystems has a long history in the Nordic region starting in the late 19th century (e.g., Rosenvinge, 1893; Ostenfeld, 1908; Waern, 1952) and shows a marked increase in research output over the past four decades to current levels of about 50 publications per year, as assessed by a bibliometric survey (Figure S1; Table S2). However, the peer-reviewed literature includes only a limited and recent focus on BC and has largely been conducted from a national angle. A first joint effort to quantify the distribution, biomass and C-storage potential of seagrass meadows (dominated by eelgrass, Zostera marina), kelp forests (large canopy-forming brown macroalgae of the order Laminarians), and rockweed beds (brown macroalgae of the order Fucales) was recently supported by the Nordic Council of Ministers (Frigstad et al., 2021). But distribution maps, knowledge of status and trends, C-stocks and sequestration rates as well as knowledge of other ecosystem functions of seagrass meadows, salt marshes and macroalgal forests/beds are still incomplete. Blue carbon habitat restoration efforts are local and the information scattered.
The Nordic management of vegetated coastal habitats is related to European directives such as the Habitats Directive, Birds Directive, NATURA 2000 network of marine protected areas, Water Framework Directive, Marine Strategy Framework Directive and Nitrate Directive; regional conventions (HELCOM, OSPAR, ICES), collaboration platforms (Nordic Council of Ministers), as well as national and sub-national laws/regulations and management initiatives. However, BC aspects have received limited policy attention at the Nordic level. Recent declarations by the Nordic Council of Ministers highlight the ocean-climate nexus, the C-sink capacity of marine ecosystems, the coupling to biodiversity, and the need for Nordic collaboration (Gudbrandsson et al., 2019) to ensure that oceans and marine ecosystems are climate-resilient and sustainably managed. BC is also gaining focus at European and global levels. Hence, BC received unprecedented attention at the recent United Nation climate change conference COP26.
Here we present the state of knowledge related to Nordic BC habitats including environmental setting and policy framework, ecosystem extent and change, C-stocks and C-sequestration rates, and management status regarding protection and restoration of BC habitats. On this basis, we identify knowledge gaps and propose directions for research priorities, as well as cross-sectoral management and collaboration towards a Nordic BC-roadmap as a nature-based solution to combat multiple societal challenges. Our goal is to support Nordic management of vegetated coastal BC ecosystems to secure the multiple functions and services they provide. This contribution was initiated at the Nordic Blue Carbon Workshop with 77 participants from 18 countries, held in Copenhagen, Denmark, September 9-13, 2019, under The Blue Carbon Initiative (BCI) Scientific Working Group’s 12th annual meeting.
The Nordic Region and Its Blue Carbon Habitats
The Nordic region includes Norway, Sweden, Denmark, Finland, Iceland, the Faroe Islands, and Greenland. It covers 28 latitude degrees from Southern Denmark at 55°N to Northern Greenland at 83°N and extends across 58 longitude degrees from the west coast of Greenland in the Baffin Bay (73°E) to Finnmark, NE Norway, by the Barents Sea (31°E). The Nordic coastline is vast and is fringed by large shallow-water areas where light reaches the seafloor supporting potential growth of vegetation (Figure S2). According to Sayre et al. (2019, Table S1), the Nordic coastline is 224,087 km long, equaling about 9% of the global coastline. This estimate is based on 30 m resolution mapping (i.e., 1:60 000 scale, Tobler 1987) and therefore excludes many small islands, which leads to a strong underestimation of island-rich archipelagoes common across much of the region. Indeed, complex archipelagoes with thousands of islands are important features of the region which hosts significant areas of BC habitats. For instance, the most updated estimate for Norway includes every island and calculates more than 100,000 km of coastline, which is almost twice the estimate of Sayre et al. (2019; 53,751 km). The average depth of the Baltic Sea, which is the world’s largest estuary, is only 55 m. According to the EMODnet digital terrain model (emodnet-bathymetry.eu) at a 1/16 arc-minute resolution (which is approximately 125 m for the Nordic region), the extent of shallow areas with a water depth less than 10 m sums up to 50,330 km2 for the Nordic countries (Svalbard and Jan Mayen included in the Norwegian estimate, but Greenland excluded due to lack of coverage by EMODnet). These shallow areas constitute on average 5.7% (3.25% if Svalbard is not included) of the countries’ maritime Exclusive Economic Zone (from the VLIZ Maritime Boundaries Geodatabase), varying from <1% for Faroe Islands, Iceland, and Norway, to 5.5% for Sweden, 13% for Denmark, and 15% for Finland.
The region is characterized by physicochemical gradients with relevance to the BC habitats. In the northernmost areas, sea-ice cover affects both light availability and physical exposure and constitutes an important structuring factor, while the southernmost areas are only affected by ice during extreme winters (Figure S2). The region has a strong salinity gradient with high saline water and large tidal range along the Atlantic coastlines and near freshwater conditions with small or no tidal range in the Baltic Sea (Figure S2). Most Nordic countries, except Denmark, have large components of rocky shores (Young and Carilli, 2019). The levels of anthropogenic stressors such as land use and fishery also vary across the region, causing multi-stressor and cascading perturbations (Boström et al., 2014; Andersen et al., 2015; Reusch et al., 2018; Krause-Jensen et al., 2021). In addition, the area is strongly affected by climate change, which has led to a warming of the annual sea surface temperature of the Baltic Sea by up to 1.0°C per decade from 1990 to 2008 (BACC II author team, 2015 p. 9); this is much greater than the global average warming rate of the upper ocean of 0.11°C per decade from 1971 to 2010 (Rhein et al., 2014).
Nordic BC habitats reflect these regional environmental gradients (Figure 1). Salt marshes are common habitats along sheltered soft bottom shores and exhibit marked diversity from full marine tidal areas dominated by typical tidal marsh to brackish microtidal regions colonized by salt meadows and reed belts (Figure 1, see further details in the subsection on salt marshes). Deeper along the coastal slope, soft and sandy seafloors are colonized by meadows of seagrasses, dominated by eelgrass (Zostera marina), and increasingly mixed with other rooted vegetation and charophyte species in the more brackish regions towards the inner basins (Gulf of Bothnia) of the Baltic Sea and in the inner part of many estuaries (e.g. Boström et al., 2014; Wikström et al., 2016; Figure 1). Rocky shores are colonized by a variety of macroalgae with rockweed, particularly Fucus species dominating the shallow/tidal zone and Laminaria- and Saccharina-dominated kelp forests (Figure 1) with understory vegetation being abundant in the marine subtidal (e.g. Fredriksen et al., 2005).
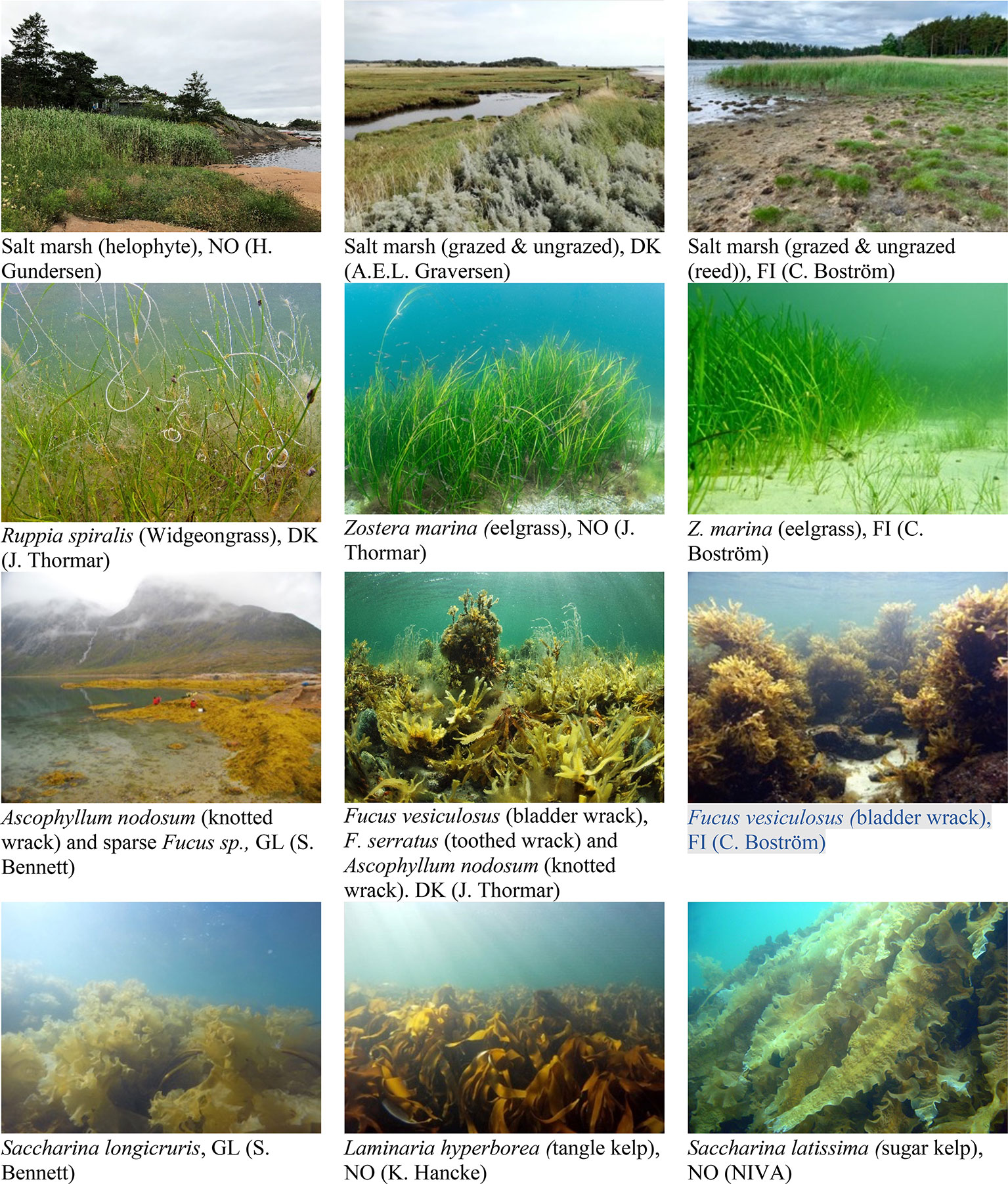
Figure 1 Examples of characteristic Nordic BC habitats: Salt marsh (1st panel), rooted vegetation such as seagrass (2nd panel), intertidal macroalgae (3rd panel), and submerged macroalgae, such as kelp forests (4th panel).
Global, Regional and National Policies Related to Nordic Blue Carbon Habitats
There is currently no specific BC policy or management strategy for BC habitats in the Nordic countries, but several policies and directives address vegetated coastal habitats and provide an important basis for more targeted and coordinated BC management. Key examples are summarized below.
Global Scale
The Glasgow Climate Pact from the 26th Conference of the parties of the UNFCCC acknowledges the C-sink capacity of marine ecosystems. Several countries have included Blue Forests in their inventories and National Determined Contributions (NDCs) under the Paris Agreement based on the voluntary “2013 supplement to the 2006 IPCC Guidelines for National Greenhouse Gas Inventories: Wetlands”, which addresses saltmarshes, seagrasses and mangroves but not macroalgae. However, none of the Nordic countries have yet included blue forests in their NDCs.
Protection of Nordic BC habitats and their services is, to some extent, also supported by global policy agreements under the Convention on Biological Diversity (CBD), the Ramsar convention for wetlands, the UNESCO Biosphere Reserves and Natural World Heritage sites, and the United Nations’ Sustainable Development Goals (e.g. UN SDG 14.5 of 10% conservation of marine and coastal areas, and UN SDG 6.6 of protection and restoration of water-related ecosystems).
Regional Scale
The European Union’s (EU) Habitat’s Directive, Birds Directive, Water Framework Directive (WFD), and Marine Strategy Framework Directive (MSFD) as well as the Nitrate directive are all relevant for Nordic BC habitats. While Sweden, Denmark and Finland are EU members, Iceland and Norway are EEA EFTA members and have adopted the WFD via the EEA agreement. To some extent, national legislation in Iceland and Norway converges with, or are indirectly impacted by, the other EU directives as well. The Faroe Islands and Greenland are neither part of EU, nor the EEA EFTA and do not have obligations relative to e.g. the WFD, but EU and Greenland reinforce their partnership after the recent adoption of the new European strategy for the Arctic region. The WFD directly supports blue carbon habitats by defining the goal of good ecological status for coastal vegetation as a situation when “most disturbance sensitive macroalgal and angiosperm taxa associated with undisturbed conditions are present and the level of macroalgal cover and angiosperm abundance show slight signs of disturbance” (WFD, 2000/60/EC). The MSFD’s requirement of “good environmental status (GES)” addresses the marine vegetation more indirectly via Descriptor 1 (biological diversity), Descriptor 4 (food webs) and Descriptor 6 (seafloor integrity). The WFD and the MSFD are closely connected; for example, marine vegetation and other so called “quality elements” targeted by the WFD are protected by management measures such as reduced eutrophication, and therefore also contributes to achieving GES within the MSFD with respect to several Descriptors.
At the regional level, the Baltic Sea Action Plan (2021) by the Helsinki Commission (HELCOM) also considers how mitigation by natural blue carbon processes can be maximized, but has no legal implications. HELCOM also defines eelgrass habitats and several macroalgal habitats in the Baltic Sea as being under threat and highlights the need for protection against e.g., eutrophication and anchoring (https://helcom.fi/media/documents/HELCOM-Red-List-Biotope-Information-Sheets-BIS.pdf). Moreover, in the North-East Atlantic, the inclusion of eelgrass beds in the OSPAR Convention’s list of threatened species has been followed up by OSPAR Recommendation 2012/4 on furthering the protection and conservation of eelgrass beds (OSPAR 12/22/1, Annex 13). Greenland also accedes to the OSPAR. Moreover, the Arctic Council, with participants from several Nordic states (i.e. the Kingdom of Denmark including Greenland and Faroe Islands, as well as Iceland, Norway, Sweden and Finland), coordinates marine activities via working groups on the “Arctic monitoring and assessment program”, “Protection of the Arctic marine Environment”, and the biodiversity working group of the Arctic Council: Conservation of Arctic Flora and Fauna (CAFF) and their Circumpolar Biodiversity Monitoring Programs (CMBP) on coastal and marine ecosystems. All these initiatives also to some extent address Nordic BC habitats.
National Scale
National management of BC habitats in EU member states must as a minimum fulfill the EU requirements and may also involve supplementary measures, which are treated in more detail in the management section (see below).
Distribution Area and Dynamics of Nordic BLUE CARBON Habitats
The total C-stocks and sequestration of coastal vegetation is connected to their area distribution. For some habitat types, like eelgrass meadows, the Nordic region has experienced major declines over the past century (Boström et al., 2014). For other BC habitats, like the kelp forests, the picture is more complex (Araújo et al., 2016). This section summarizes, by habitat type and country, the current knowledge on the distribution area of Nordic BC habitats (Table 1 and Figure 2A) and major changes over the past century.
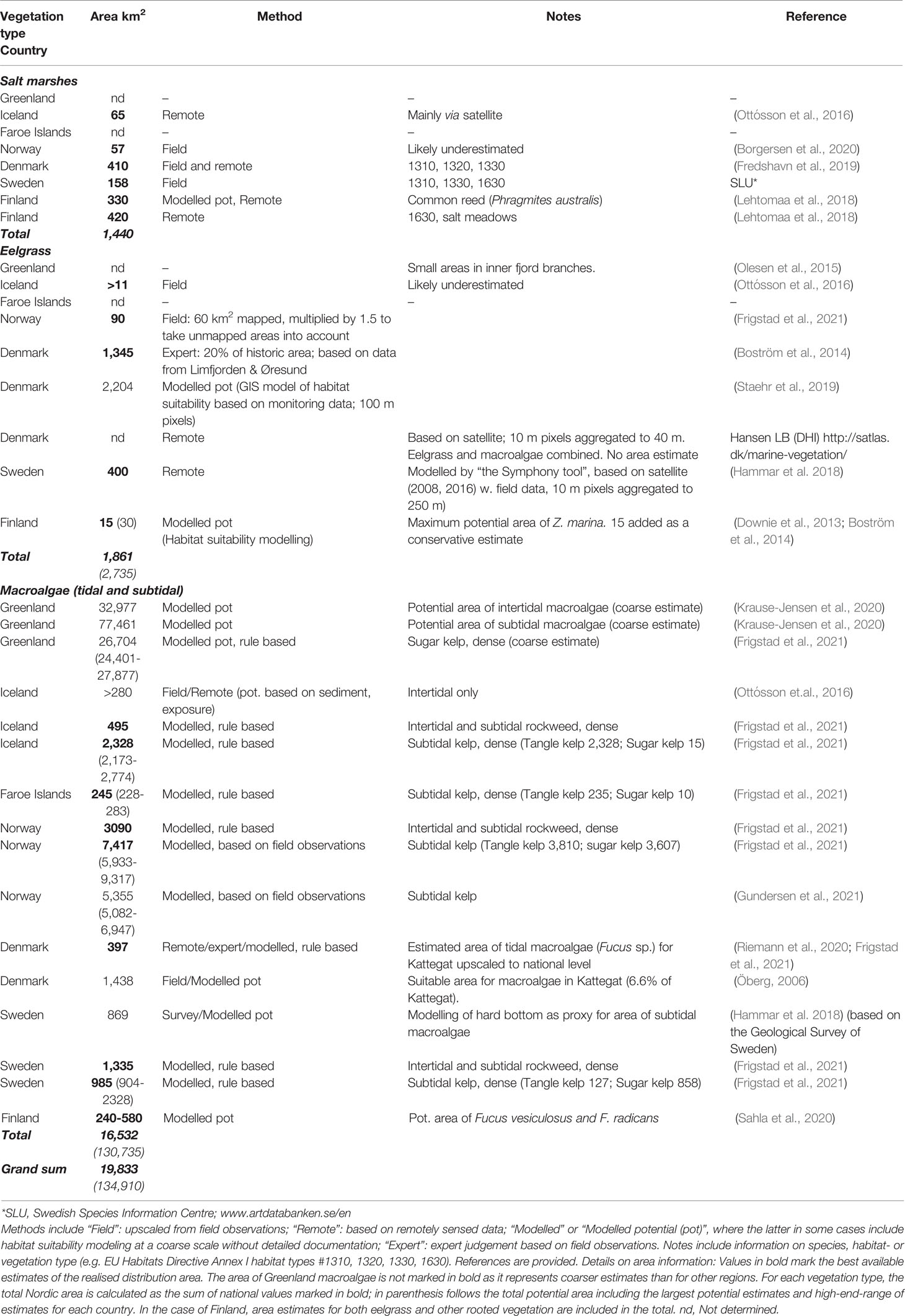
Table 1 Area estimates (km2) of coastal vegetated habitats (salt marshes, eelgrass and other rooted vegetation, macroalgae) across the Nordic region.
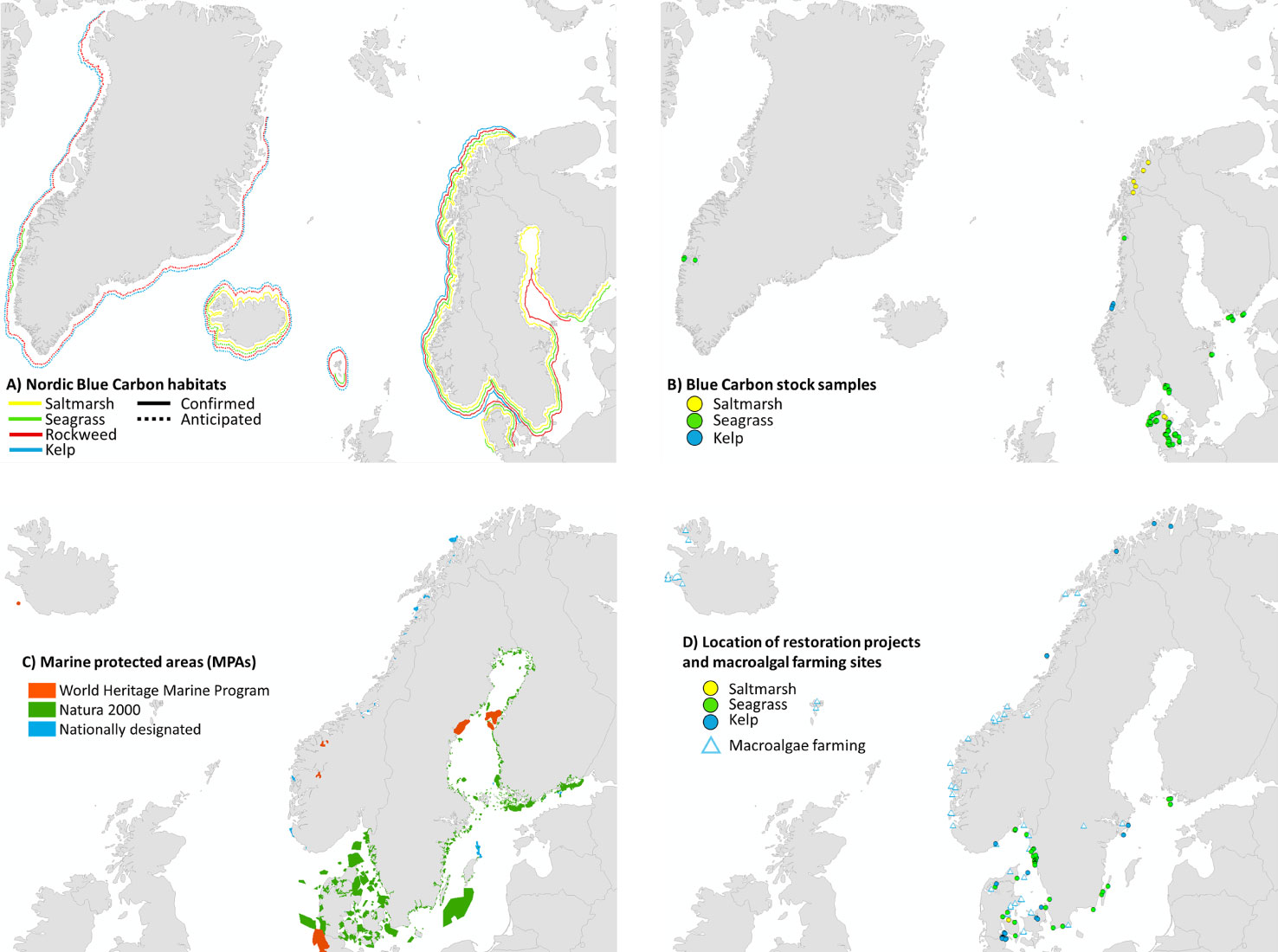
Figure 2 Overview of available information on area distribution of Nordic BC habitats (Svalbard not included). (A) Occurrence of confirmed (solid lines) and anticipated (dotted lines) Nordic BC habitats (salt marshes, eelgrass, littoral and sublittoral macroalgae) (details in Table 1); for Greenland, there are several confirmed macroalgal habitats along especially the west coast, and eelgrass habitats in the Nuuk fjordsystem, although only a coarse estimate of area distribution is available. (B) Sites where C-stocks/sequestration have been assessed. (C) Location of Marine Protected Areas (MPA’s; including Marine World Heritage sites, Natura 2000 sites and nationally designated MPAs). (D) Location of restoration projects (circles) and macroalgal farming sites (triangles, Araújo et al. 2021, from EMODNet) across the Nordic countries. Map from Google Earth, symbols from https://ian.umces.edu/imagelibrary/. See Table S5 (C-stocks) and Table S6 (restoration sites) for detailed site information.
Most Nordic countries conduct regular monitoring of BC habitats to document status and trends in relation to e.g. the Water framework directive and national programs (summarized in Table S3). However, except for the area of salt marshes, which is directly targeted by Habitats Directive monitoring, the monitored parameters are not necessarily related to the area distribution of the habitats, which is reflected in large knowledge gaps on BC habitat maps. For example, the Danish and Swedish national marine monitoring programs focuses on the depth limit and cover of eelgrass meadows and the cover of macroalgal communities (Riemann et al., 2016, Hammar et al. 2018) and the Greenland Ecosystem Monitoring (www.g-e-m.dk) focuses on the growth rate of kelps and rockweed as well as local depth distribution patterns. Norwegian coastal monitoring programs include regular measurements of the lower growth limit, coverage and status (e.g. the coverage of filamentous algae) for eelgrass and macroalgae on a fixed network of stations along the coast (Walday et al., 2020) and additional kelp properties as part of the kelp harvesting strategy (Steen et al., 2016).
Salt Marshes
The Nordic region supports a range of habitat types that we here jointly refer to as “salt marshes” although they may also fit under terms such as “salt meadows”,” coastal meadows”, “tidal marshes” or “reed belts”. They are dominated by herbs, grasses or low shrubs that are periodically inundated or saturated by tidal water (Adam, 1990; IPCC, 2014; Evans and Roekaerts, 2019). The use of different names for salt marshes and their heterogenous appearance make it challenging to get an overview of their total distribution. Comparison of these habitat across countries is also complicated by the existence of various national and international classification systems such as the European Nature Information System (EUNIS) in the EU (Evans and Roekaerts, 2019), the Nature in Norway (NiN) system in Norway (Borgersen et al., 2020; Halvorsen et al., 2020) and HELCOM for the Baltic Sea (Helcom, 2013) (details in Table S4).
There are strong gradients in “salt marsh” plant communities in the Nordic region related to gradients in tidal regime, salinity, and climate factors. A key management practice, grazing, which is often implemented to stimulate biodiversity, also markedly affect the marshes. Nordic EU member states map salt marshes as part of the Natura 2000 network, and Atlantic coastal meadows are categorized as vulnerable in the European Red List of Habitats (Janssen and Rodwell, 2016). Mapping is also taking place as part of national programs. Below is an overview of area information available for these habitats in the Nordic region. The European-funded project “NordSalt” (2021-2024, www.sdu.dk/en/forskning/nordsalt) will expand the information on salt marshes and their C-stocks and sequestration in the Nordic region.
Greenland
There is no overview of area distribution or changes in distribution of salt marshes at the Greenland scale. However, some local distribution studies exist (e.g., Glooschenko et al., 1993; Lepping and Daniëls, 2007; Bültmann and Daniëls, 2013), and salt marsh sediments have also been studied as archives of recent relative sea level change (Jensen et al., 2006; Woodroffe and Long, 2009; Long et al., 2012).
Iceland
Salt marshes in Iceland are classified into two habitat types. One is characterized by Puccinellia maritima (Atlantic lower shore communities under the EU habitat type 1330). The other is classified by Carex species (Icelandic Carex lyngbyei salt meadows). The latter is not accepted under EUNIS but proposed as a sub-type of Atlantic black sedge salt meadows that falls under Annex I at a higher level (see A2.5 – https://eunis.eea.europa.eu/habitats/2931). Together, the habitat types cover approximately 65 km2, with the majority characterized by P. maritima (Ottósson et al., 2016). The habitat mapping was done mainly by satellite image interpretations so estimation errors can be relatively high, especially for Carex habitats due to indistinct differences between similar habitats. No national monitoring of salt marshes exists in Iceland and long-term changes and dynamics are therefore unknown.
Faroe Islands
No overviews of distribution area or dynamics are available for the Faroe Islands (https://kort.foroyakort.fo/kort/). Salt marsh species such as Carex lyngbyei, Glyceria fluitans, G. maxima and Puccinellia maritima occur in the Faroe Islands but are all reported as very rare (reported 1–10 times) or rare (reported 11–25 times) (Jóhansen et al., 2000) indicating that salt marsh areas are negligeable in extent.
Norway
Norway has no systematic mapping program for salt marshes, but some mapping efforts have been completed, e.g. in connection to mapping RAMSAR areas. Typical species in Norway are Carex paleacea, C. vacillans, Glyceria fluitans, G. maxima, Phragmites australis, Schoenoplectus lacustris, S. tabernaemontani and Bolboschoenus maritimus. Currently, 606 occurrences of salt marshes have been recorded in Norway with a total area of 57 km2 (Borgersen et al., 2020). This is most likely an underestimate due to the lack of systematic mapping. The habitat is considered as of “least concern” in Norway’s red list for habitats (Gundersen et al., 2018).
Denmark
Danish salt marshes are represented by the EU habitat types 1310, 1320 and 1330 (Table S4), with 1330 being the most widely distributed and categorized into two subtypes, salt marsh and salt swamp, depending on whether it is being grazed or not. The grazed plant communities are dominated by halophytic grasses and herbs, whereas the non-grazed ones are dominated by reeds such as Phragmites australis, Schoenoplectus maritimus and Schoenoplectus tabernaemontani. This division is the result of management practices with a long history of grazing preventing overgrowth by reed. The Wadden Sea has the largest tidal range among Danish salt marshes and includes typical tidal Spartina spp. salt marshes (EU habitat type 1320). Many of these marshes were formed as part of land reclamation efforts (Jakobsen, 1954). All habitat types are monitored and mapped every six years under the Danish monitoring program NOVANA since 2007, with the most recent estimate representing a total of 410 km2 (sum of the area of the EU habitat types 1310, 1320 and 1330; Fredshavn et al., 2019; Table 1, https://naturdata.miljoeportal.dk/). The saltmarsh area is estimated to have been twice as large as 150-200 years ago, with the loss caused primarily by expansion of agriculture (Vestergaard, 2000). EU habitat types 1310 and 1330 are categorized as having an unfavorable preservation status, the latter showing decline over the past 12 years, while 1320 has a favorable status (Fredshavn et al., 2019, Table S4).
Sweden
The extent of Swedish salt marshes is estimated at 158 km2 based on data and information from the report of conservation status of habitats and species that is provided by the implementation of the EU Habitats Directive (under Article 17), which is synthesized and published every six years by the Swedish Species Information Centre, ArtDatabanken. The estimates include the EU habitat types 1310, 1330 and 1630 (Table S4), which are all categorized as having bad conservation status.
Finland
The majority of Finnish salt marshes belongs to the EU habitat type 1630 (Boreal Baltic coastal meadows). There are no overviews for habitat types 1310, 1320 or 1330 (Table S4), but 1330 is part of a recent classification (Pätsch et al., 2019). Traditionally managed (grazing, mowing) salt marshes have been classified (by IUCN) and their status assessed (improving, declining, stable) in the national red list of threatened habitat types (Lehtomaa et al., 2018). These marshes belong to the habitat type 1630 and are currently classified into six types: seashore meadows Eleocharis parvula-E. acicularis, Eleocharis palustris-Schoenoplectus tabernaemontani-Bolboschoenus maritimus, tall-sedge seashore meadows, low-graminoid seashore meadows, tall seashore meadows and salt patches. Of a total of about 6900 km2 of traditionally managed salt meadows in the 18th century, only about 420 km2 exist today (Lehtomaa et al., 2018). Reed belts have been favored by a dramatic decline in grazing by livestock as well as by eutrophication so the area of completely or partly preserved meadows of the habitat type 1630 is only 62 km2, of which about 20 km2 is overgrown by reed. Hence, the habitat type 1630 is now classified as critically endangered (Schulman et al., 2008). It is estimated that about 121 km2 of this habitat type can potentially be restored, and there are ongoing management initiatives (including grazing). Coastal reed belts with Phragmites australis, Schoenoplectus and Bolboschoenus maritimus or Typha are additional potential BC sinks not belonging to the habitat type 1630.
Seagrasses and Other Rooted Submerged Vegetation
In the Nordic region, there are two true seagrass species, eelgrass (Zostera marina) and dwarf eelgrass (Z. noltei). Eelgrass is by far the most dominant and subject to a long record of research, particularly in Denmark (Boström et al., 2014; Krause-Jensen et al., 2021). Other rooted vegetation, such as species of the genera Ruppia, Zannichelia and Potamogeton, may also occur in brackish areas. For the purposes of this paper, when the term seagrass or eelgrass is used for the Nordic region, we are referring to primarily eelgrass, occasionally including other species of rooted vegetation. Eelgrass meadows were historically very extended but experienced major declines in the 1930s due to the wasting disease, which decimated the North-Atlantic eelgrass meadows, and more recent declines due to multiple stressors (Krause-Jensen et al., 2021).
Greenland
Recent studies of eelgrass in the Nuuk fjord system at 64 °N show that the meadows are confined to inner, protected fjord branches with sandy seafloor where summer temperatures are relatively high (up to 13°C), although a sparse meadow grows in an area with maximum summer temperatures of 8°C (Olesen et al., 2015). These meadows support biomasses as high as those at lower latitudes but have lower productivity (Olesen et al., 2015). There is no assessment of the overall distribution area, but it is relatively small, limited by both availability of suitable sandy/soft seafloor in shallow protected settings and by low water temperatures (Olesen et al., 2015). Dating of sediments in eelgrass meadows in the Nuuk fjord system along with analyses of the origin of organic matter in these sediments, nevertheless, suggest that eelgrass has been expanding in these locations over the past century (Marbà et al., 2018).
Iceland
Macrovegetation on littoral sediments include eelgrass beds, which are most common along the west coast and in lagoons in the south-east while rare elsewhere (Boström et al., 2014). They cover roughly 1% (11 km2) of the coast (Ottósson et al., 2016), but this is likely an underestimate as the distribution area has only been partially explored. Lack of monitoring and mapping efforts prevent a detailed account of the status and trends.
Faroe Islands
Eelgrass is very rare in Faroe Islands. It has been reported less than 10 times (Jóhansen et al., 2000) but with no recent observations. The extent is therefore considered negligeable with no overview of the distribution area or trend. Eelgrass occurs in one of the fjords on the southernmost island, Suðuroy, where Ostenfeld (1905-08) already a century ago commented that this fjord was likely the only place where the species occurred.
Norway
Eelgrass meadows are the most common and widespread habitat-building species on soft seafloor in Norwegian fjords and bays. The largest occurrences of eelgrass meadows have been systematically mapped by the Norwegian National program for mapping of coastal biodiversity (Bekkby et al., 2013), and are found in sheltered and moderately wave exposed areas all the way from the Swedish border in southern Norway to the Barents Sea region in Finnmark. More than 3000 eelgrass meadows have been identified in Norway, covering 60 km2 in total (Bekkby et al., 2013). Following the “red list” protocol (Gundersen et al., 2018), this area was multiplied by a factor of 1.5 to include meadows assumed to be present but not recorded, giving an estimate of 90 km2 (Frigstad et al., 2021). The habitat is not considered threatened in Norway’s red list for habitats (from 2018), but the status and trends in the North Sea in particular are largely unknown and highly debated, as the methods used for monitoring do not capture changes in meadow size and lower growth limit (Gundersen et al., 2018). Degradation of the seagrass meadows in the Oslofjord has been documented (Dahl et al., 2008; Espeland and Knutsen, 2014).
Denmark
Denmark is the Nordic ‘hotspot’ for eelgrass due to the extensive coastline with gently sloping sandy shores in protected settings of intermediate salinity. The national Danish monitoring program assesses annually the cover and depth distribution of eelgrass across the country along transect lines from the shore to the deepest occurrence. Area surveys are not part of the monitoring program, except for the Wadden Sea eelgrass meadows. A first nationwide mapping of the submerged coastal vegetation (eelgrass, other rooted vegetation and macroalgae) has been produced based on optical satellite data from 2018, using advanced radiative transfer modelling and machine learning techniques. The map has 10 m resolution and represents depths down to 4–10 m, depending on local water clarity (http://satlas.dk/marine-vegetation/), but does not distinguish between eelgrass and macroalgae and provides yet no information on the distribution area. However, the potential distribution area of eelgrass in Danish coastal waters has been quantified at 2,204 km2 based on a GIS modelling of where habitat requirements are fulfilled (Stæhr et al., 2019). This is about a third of the historic distribution area quantified at 6,726 km2 around year 1900 (Petersen, 1914). Overviews for two key distribution areas (the Limfjord and Øresund) suggest that the current actual distribution area of eelgrass is probably only about 20% of the historic area, i.e. about 1,345 km2 (Boström et al., 2014). Century-long records reveal shifting challenges to eelgrass over time with the wasting disease decimating the populations in the 1930s, eutrophication peaking in the 1980s causing additional decline, and bottom-trawling and warming further limiting the recovery during the past decades (Krause-Jensen et al., 2021).
Sweden
The area distribution of eelgrass in Swedish coastal waters is assessed at 400 km2 using a model based on satellite remote sensing images (2008 and 2016) and associated ground truthing (>6000 sites) for field validation (the Symphony tool, Hammar et al. 2018) (Table 1). The spatial model described the probability of occurrence of seagrass (in 10 x 10 m pixels, aggregated into 250 x 250 m pixels); the probability level was set at 0.8, which means that there is at least 80% probability of finding the focal habitat in a pixel. The modelled coastal area encompasses primarily eelgrass (Z. marina), but also to some extent widgeon grass (Ruppia spp.) and occasionally some freshwater green algal species (principally along inner margins of Z. marina) (Boström et al., 2003). The model has been used efficiently in recent spatial risk assessment of global change impacts on eelgrass in Sweden (Perry et al., 2020). Satellite remote sensing has been shown useful to assess eelgrass coverage down to about 5-6 meters depth on the Swedish Skagerrak coast (Envall and Isaksson, 2012; Lundén and Gullström, 2013). In general, the cover of soft-bottom vegetation decreases with increasing total nitrogen concentrations and salinity (Wickström et al., 2016). Eelgrass meadows along the Swedish west coast experienced major declines over the period 1980s-2000s (Baden et al., 2003; Nyqvist et al., 2009) due to the combination of eutrophication and overfishing (Moksnes et al., 2008; Baden et al., 2010), and climate change is emerging as an additional stressor with increases in sea surface temperature, ocean acidification and wind-driven turbid conditions likely to cause interactive negative effects in risk areas in southern Sweden by the mid-end century (Perry et al., 2019; Perry et al., 2020).
Finland
There is no comprehensive area estimate of eelgrass coverage in Finland, but habitat suitability models suggest a maximum of 30 km2 (Downie et al., 2013; Boström et al., 2014). As no national monitoring of eelgrass meadows exists, there are only few examples of long-term dynamics of Finnish eelgrass meadows. The available data suggest that these systems have been stable over time i.e. 1970-1990 (Boström et al., 2002). Finnish eelgrass meadows are often multi-specific, and eelgrass occurs here at the physiological boundary and is thus particularly vulnerable. Individual meadows are small, isolated clones with limited geneflow typically reaching over thousand years of age (Reusch et al., 1999; Reusch and Boström, 2011). The overall low reservoir of genetic diversity in the northern Baltic Sea combined with extremely limited sexual reproduction and inbreeding typically lead to reduced fitness (Reusch et al., 1999; Olsen et al., 2004). Thus, these populations are in urgent need of special conservation efforts as they lack potential for rapid adaptation and are likely to go extinct under extreme climate events.
Other submerged aquatic vegetation habitats that include plants of freshwater and/or marine origin are potentially more important BC habitats in Finland. These have been classified and their status assessed (Lehtomaa et al., 2018). They include habitats dominated by Potamogeton and/or Stuckenia pectinata, Ranunculus spp., Zannichellia spp. and/or Ruppia spp., Myriophyllum spicatum and/or M. sibiricum, and exposed and sheltered habitats characterized by Charales and Najas marina.
Macroalgae – Intertidal and Subtidal
Macroalgae represent the largest BC habitat of the Nordic region with an estimated coverage of >10 000 km2 (excluding area estimates from Greenland, which are very uncertain, Frigstad et al., 2021). Intertidal macroalgal habitats, largely defined by tidal range and the occurrence of hard substratum, are most conspicuous along the coasts of Greenland, Iceland, Faroe Islands, Norway and SW Sweden. The microtidal rocky south- and east coast of Sweden and the Finnish coast are also rich in habitats of Fucus sp. (Rinne & Salovius-Laurén, 2020; Sahla et al., 2020), which colonize the low-saline subtidal in addition to the intertidal zone (Torn et al., 2006). Here they penetrate deeper than in full marine settings because of reduced competition from other macroalgae (Nielsen et al., 1995; Middelboe et al., 1997). In contrast, most of the Danish coastline has only scattered stones and narrow tidal range and therefore limited distribution of intertidal macroalgal communities. The occurrence of subtidal macroalgae largely follows the pattern described for the intertidal habitats, i.e. dominating the rocky coastlines, although also growing on stone reefs, e.g. in the Kattegat. Sandy seafloors may also hold macroalgae such as Charales, floating macroalgae as well as macroalgal communities on scattered stones and shells. Overall, the macroalgal community of the region is characterized by steep declines in species diversity along the Baltic salinity gradient (Nielsen et al., 1995).
Greenland
Macroalgae occur along most of Greenland’s coastline, but distribution records are relatively sparse, especially in the northernmost regions (Krause-Jensen et al., 2020). The depth limit and width of the belt of submerged macroalgae, dominated by kelps, increase from north towards south as the length of the open water period increases and more of the surface light potentially reaches the seafloor (Krause-Jensen et al., 2012). The deepest occurrence of kelps (> 60 m depth) is reported in clear waters along open coasts and offshore islands (Krause-Jensen et al., 2019). Intertidal macroalgae are also abundant along the Greenland coast, with potential for high biomasses even in northern regions (Høgslund et al., 2014; Ørberg et al., 2018; Sejr et al., 2021; Thyrring et al., 2021). Whereas no detailed mapping of macroalgae in Greenland exists, a first, coarse, spatial distribution model suggests a potential intertidal macroalgal distribution area of about 33,000 km2 and a subtidal distribution area of 77,500 km2 (Krause-Jensen et al., 2020). Also, Frigstad et al. (2021) estimated the total coverage of dense Saccharina latissima (sugar kelp) forests in Greenland to be 26,700 km2 in a Nordic spatial model but emphasized that this is an undocumented estimate with high uncertainties due to unknown effects of sea ice cover, exposure, and substrate type. Macroalgal distribution and production is hypothesized to increase in parallel with increasing water temperatures and declining sea ice cover (Krause-Jensen et al., 2020).
Iceland
Rocky shores dominated by macroalgae cover 28% (280 km2) of the intertidal in Iceland (Ottósson et al., 2016). Sheltered intertidal areas that are dominated by A. nodosum cover >7% of the total intertidal area and >25% of all low to moderate energy littoral rocky shores. Breiðafjörður in West Iceland accounts for about 70% of intertidal areas (50 out of 70 km2), dominated by A. nodosum according to habitat mapping done in 2012-2014 (vistgerdakort.ni.is, 2018). More recent, in-depth studies expand this estimate to 91-107 km2 (Gunnarsson et al., 2019). Frigstad et al. (2021) estimated a total coverage of 495 km2 for rockweed and 2,328 km2 for kelps, dominated by Laminaria hyperborea (tangle kelp), based on a spatial model covering the Nordic countries. No national monitoring of macroalgae exists so long-term trends and dynamics are unknown.
Faroe Islands
Kelps are widespread on the rocky shores of the Faroe Islands, and data from the FARCOS project in the 1990s (Bruntse et al., 1999) were recently used to model the potential kelp distribution around the Faroe Islands, predicting a coverage of 10 km2 for sugar kelp and 235 km2 for tangle kelp (Table 1, Frigstad et al., 2021). No estimates exist for the coverage of macroalgae in the littoral zone of the Faroe Islands.
Norway
Rockweeds cover the intertidal shores and the shallow subtidal areas along almost the entire Norwegian coast, with a total coverage estimated (modelled) to be 3,090 km2 (Frigstad et al., 2021). Tangle kelp and sugar kelp are the two dominant species that form large subtidal kelp forests along the Norwegian coast. Tangle kelp is found in the wave-exposed areas and has been mapped systematically by the National program since 2007 (Bekkby et al., 2013), estimated to cover 3,810 km2 (Frigstad et al., 2021). Sugar kelp forests colonize more sheltered areas, often in fjords, bays and in inner parts of archipelagos, and are mapped less systematically. These forests have been estimated to cover 3,607 km2 by Frigstad et al. (2021) and 5,355 km2 by Gundersen et al. (2021). Both estimates are adjusted for destructive sea urchin grazing in northern Norway (additionally 1592 km2 of kelp forest is considered to be grazed by urchins, Gundersen et al., 2021). Tangle kelp forests are in the Norwegian “Red list” for habitats considered to be “near threatened” in northern Norway due to the intensive grazing by the green sea urchin Strongylocentrotus droebachiensis (Rinde et al., 2014). Sugar kelp is listed as endangered due to sea urchin grazing in the north and due to a combination of eutrophication and increasing frequency and intensity of marine heat waves in the south of Norway (Gundersen et al., 2018; Filbee-Dexter et al., 2020). For sugar kelp in southern Norway, a combination of increased water temperature and increased riverine inputs of terrestrial organic matter (increased light attenuation and sedimentation) has negatively affected the lower growth depth limit over the last decades (Frigstad et al., 2018). This trend is leading to a decrease in macroalgal area coverage, decreased primary production, and a subsequent decrease in macroalgal ecosystem services, including macroalgae-driven C-sequestration (Filbee-Dexter and Wernberg, 2018; Wernberg et al., 2019).
Denmark
Although most of the Danish seafloor is soft, macroalgae occur on scattered stones along the shores as well as on offshore stone reefs. As a component of the national Danish monitoring program, the cover and species composition of macroalgal communities are assessed nationwide along shoreline depth gradients representing hard substratum, as well as on offshore stone reefs, but a map of overall macroalgal distribution in Denmark is lacking. However, recently, the area extent of Fucus sp., which is dominant in the tidal zone, was quantified for the Kattegat coast at about 54 km2 based on analysis of aerial photos supported by monitoring data and drone/field surveys in test areas (Riemann et al., 2020). This area was upscaled to an estimated distribution area of intertidal algae in Denmark at 397 km2, assuming similar area extent of Fucus per km of coastline as for the Kattegat (Frigstad et al., 2021). The distribution area of subtidal macroalgae in the Kattegat has been assessed at 1438 km2 based on monitoring data, seafloor properties and modelling (Öberg, 2006). Large uncertainties in the area of hard substratum have so far prevented a reliable updated modeling of the subtidal macroalgal area (Frigstad et al., 2021).
Several stress factors, varying in space and time, affect Danish macroalgae. Vast macroalgal habitats have been lost due to extraction of stones for construction, a conservative estimate suggests that a total of 40 km2 of exposed stone surface was removed from stone reefs in coastal Danish waters in the second half on the 20th Century (Dahl et al., 2003) before the practice was banned in 2010 (Dahl et al., 2016a). Bottom trawling for mussels and fish has added to the loss of stones. Eutrophication further limits the cover and diversity of both coastal and offshore stone reef macroalgal communities through light limitation and overgrowth by opportunistic species (Riemann et al., 2016).
Sweden
The area of hard bottom within the photic zone was modelled at 869 km2 and used as a proxy for the extent of subtidal macroalgae (Hammar et al. 2018). The hard bottom model is based on geological mapping surveys conducted by the Geological Survey of Sweden (SGU). The model was set to 0.8, which means that there is at least 80% probability of finding the focal habitat in a pixel. In the Nordic model of Frigstad et al. (2021), the total coverage of tangle kelp and sugar kelp in Sweden were estimated at 127 and 858 km2, respectively, all at the Swedish west coast. The differences in area estimates for subtidal macroalgae by the two models can e.g. be due to different resolution of the models. For Fucus sp., the total coverage in Sweden was estimated at 1335 km2 using the same approach as for Denmark, assuming similar area extent per km of coastline as for the Kattegat (Riemann et al., 2020), excluding the northern east coast of Sweden with known low distributions of Fucus sp. (Frigstad et al., 2021).
Until today, there are no comprehensive overall estimates of macroalgal distribution or change in cover in Swedish marine waters. But long-term studies on depth distribution (based on annual surveys) of macroalgal zonation, cover and community composition are available (e.g., Kautsky et al., 1986; Karlsson, 2007; Hammar et al. 2018) as well as scattered spatial surveys in localized areas (e.g., Blomqvist and Olsson, 2007; Gullström et al., 2009). A harmonized nation-wide macroalgae monitoring program is, however, ongoing since 2019, which aims to assess ecological status and spatiotemporal distribution of macroalgae on the west- and east coasts of Sweden (led by SWaM and following methodology in Lindegarth et al., 2016).
Finland
The Finnish Inventory Programme for the Underwater Marine Environment (VELMU) has systematically made inventories of macroalgae since 2011. Macroalgal monitoring started in 1993 and the monitoring of the lower depth limit of Fucus started in 2000. Based on habitat suitability modelling (GAM, EADM), the Finnish coastline (including Åland Islands) today supports about 240-580 km2 of Fucus (including F. radicans) compared to >1000 km2 in 1900 (Sahla et al., 2020).
Overall
The review identified the known area extent of BC habitats in the Nordic region, which was 1441 km2 for salt marshes, 1,861 km2 (potentially >2,735 km2) for seagrasses (mainly eelgrass) and 16,532 km2 (potentially 130,735 km2, including coarse Greenland estimates) for macroalgae. This sums up to 19,833 (potentially as much as 134,910 km2) of blue forests in the Nordic region (Table 1 and Figure 2A). Although the numbers are associated with considerable uncertainty and contain major knowledge gaps, the area of Nordic marine forests is substantial. The overview reflects the increasing difficulty of mapping the habitats as the water depth increases and conceals the vegetation. Hence, actual distribution maps are very limited and the difference between documented and potential areas is huge for macroalgal habitats, which extend deepest among the BC habitats. Especially for Greenland, Faroe Island and Iceland, there is very limited documentation of area extent of BC habitats. The various estimates also represent major differences in terms of quantification of presence/absence versus dense vegetation, key macroalgal species (e.g. sugar kelp) versus overall macroalgal cover and regarding the pixel size and scale of the survey. Only unified mapping approaches across the Nordic region, such as the one carried out by Frigstad et al. (2021) offer direct comparison across part of the region but depend on further data input for data poor regions, such as Greenland. Whereas there has been very limited Nordic focus on particularly salt marshes in the BC context, Nordic EU member states quantify salt marsh areas as part of the requirements of the EU habitats directive.
In addition to the above-listed areas, EU member states report the areas and status/trends of the EU habitats directive’s marine habitat types, several of which contain meadows of eelgrass and other rooted vegetation or macroalgae beds. However, in opposition to the directive’s continental habitat types, the marine habitat types are defined based on geomorphology (e.g. “Estuaries, habitat type 1130”, “Coastal lagoons, habitat type 1150” and “Large shallow inlets and bays, habitat type 1160”) and, hence, unfortunately, do not specifically target the vegetated habitats.
It is important to underline that the above estimates of the area of Nordic BC-habitats do not provide information on the extent of manageable areas with regard to restoration or protection against further loss and, hence, the extent of area that is relevant for climate change mitigation policies.
C-Stocks and Sequestration in Nordic Blue Carbon Habitats
Salt Marshes
Pioneering data on C-stocks and sequestration rates in Nordic salt marsh sediments are available from Denmark and Norway (Figure 2B and Table S5). C-stocks are 4228-8178 g Corg m-2 for the upper 43 cm sediment in three microtidal salt marshes along the east coast of Jutland (Table 2, Graversen et al., 2022). C-sequestration rates at the same sites are 17-45 g Corg m-2 yr-1 (Table 3, Graversen et al., 2022). Sequestration rates of salt marshes in the German Wadden Sea, likely also representative for the Danish Wadden Sea, are quantified at 149 g C m-2 yr-1 over a 50 yr period and 112 g C m-2 yr-1 over longer term (Mueller et al., 2019), i.e. higher than those for Danish microtidal salt marshes. The estimates include the sequestration of both autochthonous and allochthonous carbon. In Arctic Norway, C-sequestration rates of five salt marshes are 19-603 g C m-2 yr-1, increasing e.g. with longer growing seasons; C-stocks in the corresponding sediments are limited to 367-1379 g m-2, resulting from thin sediments, e.g. due to isostatic uplift (Ward, 2020). In addition to the C-sequestration within the habitats, there is also a scope for exported saltmarsh detritus ending up in other sink habitats such as seagrass meadows.
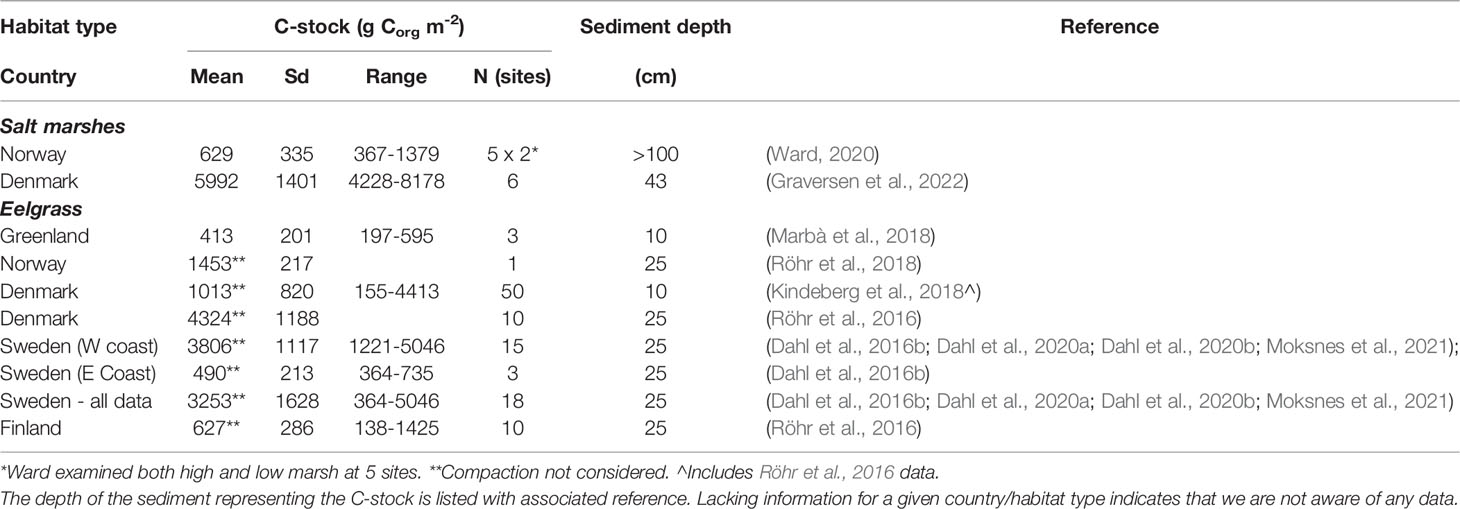
Table 2 Sediment organic C-stocks of Nordic BC habitats with indication of mean levels, standard deviation (sd), range, and number of observations (n).
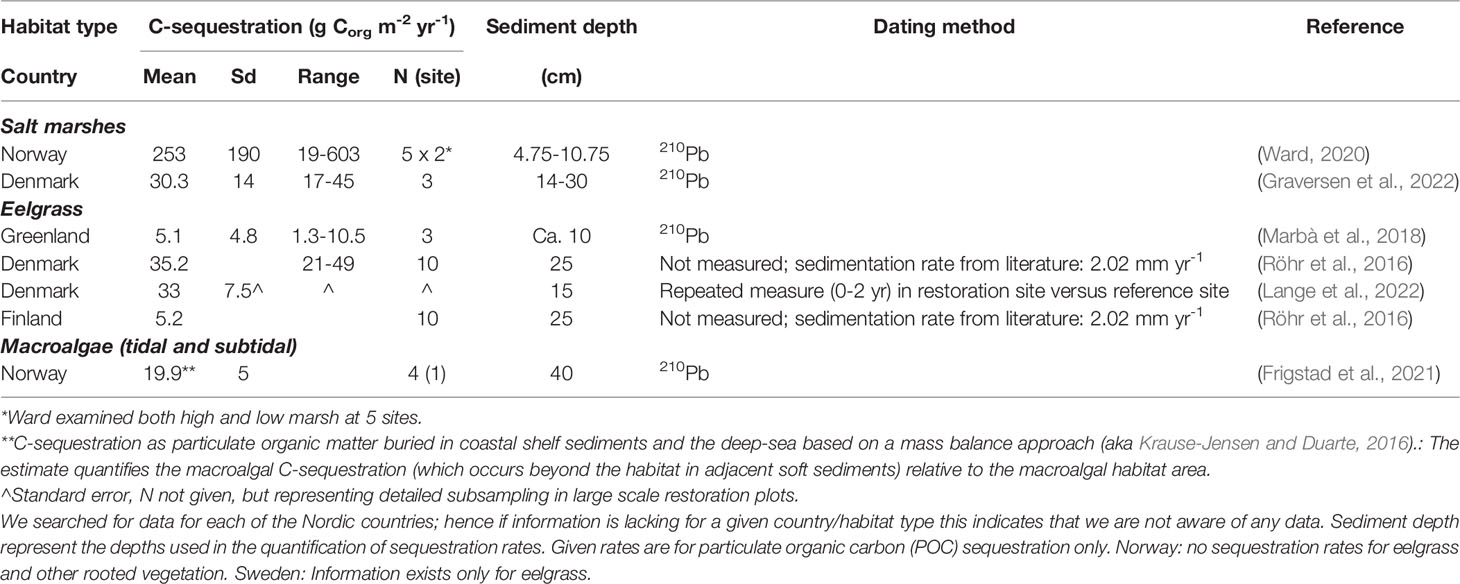
Table 3 Sediment C-sequestration rates for Nordic BC habitats with indication of mean levels, standard deviation (sd), range and number of observations (n).
The evidence is still insufficient to provide a reasonable Nordic estimate of saltmarsh C-stocks and sequestration as the few data points represent only about 10 sites spanning sediment depths of 40-100 cm and showing 5-10 fold variation between estimates; the estimates of area extent add further uncertainty. Hence, the average sediment C-stock (3311 g Corg m-2, Table 2) and C-sequestration rate (142 g Corg m-2 yr-1, Table 3) multiplied by the combined Nordic extent of salt marsh as quantified for Norway, Denmark, Sweden and Finland (1440 km2, Table 1) gives only a vague indication of the potential C-stock (4.77 Tg Corg) and C-sequestration (0.20 Tg Corg per year, i.e. 0.75 million tonnes CO2e yr-1), which need further validation.
Seagrass Meadows
Data on C-stocks in eelgrass meadows are available for sites in Greenland, Denmark, Sweden and Finland (Figure 2B and Table S5). C-stocks for the upper 10 and 25 cm sediments are 197-4413 and 364-5046 g Corg m-2, respectively, with higher stocks in the western Baltic Sea than in the eastern Baltic Sea and Greenland (Table 2, Röhr et al., 2018). The considerable variation in C-stocks both between and within regions relate to factors such as the degree of exposure and sediment characteristics with the highest stocks in sheltered settings with fine sediments (Röhr et al., 2016; Dahl et al., 2016b; Kindeberg et al., 2018; Dahl et al., 2020b).
C-sequestration in eelgrass sediments has solely been quantified for meadows in Greenland (via Pb210 dating) and over the first two years of a Danish eelgrass restoration (by repeated measure of sediment C-stocks in restored versus reference areas) (Table 3). These estimates range from an average of 5.1 g Corg m-2 yr-1 in Greenland to an average of 33 g Corg m-2 yr-1 associated with the eelgrass restoration in Denmark (Table 3). Sequestration has also been estimated for Danish and Finnish eelgrass meadows based on literature values on sediment accretion multiplied by measured C-densities (Röhr et al., 2016). In comparison with the initial sparse data from the Nordic region, a thorough recent study from the east coast of the USA reported net C-sequestration rate for eelgrass meadows (11.5 g Corg m-2 yr-1) that is compensated for the flux of greenhouse gasses (Oreska et al., 2020). Multiplying the estimated Nordic extent of eelgrass (1861 km2, Table 1) by, respectively, the average C-stock (2414 g Corg m-2, 25 cm depth, Table 2) and the net C-sequestration rate (11.5 g Corg m-2 yr-1, Oreska et al., 2020), the total sediment C-stock is approximately 4.49 Tg Corg and the sequestration rate 0.021 Tg Corg per year (corresponding to 0.08 million tonnes CO2e yr-1). As for salt marshes, these estimates are very coarse and preliminary and need further validation.
Eelgrass also supports sediment C-stocks and C-sequestration beyond the habitats (Duarte and Krause-Jensen, 2017) as first documented by eelgrass tracer studies already a century ago, which concluded that the organic matter of sediments in Danish fjords then derived almost entirely from eelgrass (Boysen-Jensen, 1914). Moreover, findings of eelgrass in ancient sediments (5600-6200 ca. BP) from Blekinge, in the SE Sweden, suggest the capacity for sequestration over Millenia (Yu et al., 2004).
Macroalgal Habitats
Most macroalgae, including kelp and rockweed, attach to hard substrates, such as rocks and stones, where organic matter does not accumulate. Macroalgae export part of their photosynthetic production as particulate and dissolved organic carbon (POC and DOC, respectively) to adjacent systems. This export supports secondary producers in the sea or on beaches (when washed ashore), while a small fraction is sequestered in C-sinks in marine sediments or the deep sea (Krause-Jensen and Duarte, 2016, Quierós et al., 2019, Ortega et al., 2020). This variety of export pathways and fates of macroalgal organic carbon makes it complex to constrain carbon budgets and sequestration rates for kelp and rockweed habitats. And because macroalgal C-sequestration in sediments occurs beyond the habitat, estimates are not directly comparable to those of sediment C-sinks in salt marshes and seagrass meadows.
In the Nordic region, the first studies documenting macroalgal export to sediment C-sinks are appearing. For northern Norway, the export rate of kelp to adjacent and off-shore sediments is quantified at levels close to the annual net kelp primary production, implying that most of the primary production is exported beyond the habitat. Part of this export supports sediment C-stocks and sequestration (Filbee-Dexter et al., 2018; Wernberg and Filbee-Dexter, 2018; Filbee-Dexter et al., 2020; Pedersen et al., 2020). For example, isotopic tracers show that macroalgae contribute to C-stocks in Danish eelgrass sediments (Thormar et al., 2016), environmental DNA (eDNA) document macroalgal C in coastal Greenland sediments (Ørberg et al., 2022), and eDNA supplemented with quantitative polymerase chain reaction (qPCR) document that macroalgae contribute to C-stocks and sequestration in coastal Norwegian sediments (Frigstad et al., 2021). An ongoing global study with Danish and Norwegian participation is quantifying the potential contribution of macroalgal farming to C-sequestration in sediments below the farms (www.oceans2050.com/seaweed). But no peer-reviewed sequestration rates of neither farmed or wild macroalgae are yet available from the Nordic region.
A first coarse, non-peer reviewed, estimate of the contribution of Nordic (excluding Greenland) kelp and rockweed habitats to C-sequestration was recently derived from the combined area of Nordic kelp forests (10,990 km2) and rockweed beds (5,556 km2), multiplied by an estimate of sequestration of Nordic macroalgal POC per macroalgal habitat area (19.9 ± 5 g C m-2 y-1, Table 3, Frigstad et al., 2021). This POC-sequestration estimate is based on a mass balance approach similar to that developed for the global estimate of macroalgal C-sequestration (Krause-Jensen and Duarte, 2016) but with Nordic data on macroalgal NPP and POC export. On this basis, the sequestration of macroalgal POC in coastal shelf sediments and the deep sea was coarsely estimated at 0.328 ± 0.082 Gg Tg C per year (corresponding to 1.18 ± 0.3 million tonnes CO2e yr-1, Frigstad et al., 2021). This first rough assessment needs further verification by peer-reviewed regional empirical data.
All in all, our review documents considerable Nordic information on sediment C-stocks of seagrass habitats, but limited information on C-sequestration rates for seagrass sediments and limited information on both sediment C-stocks and C-sequestration rates for other Nordic blue carbon habitats. While the compiled information represents sequestration of POC, DOC from BC habitats may also contribute to C-sequestration if being refractory or if exported to the deep sea (e.g. Krause-Jensen and Duarte, 2016). Quantification of this component is associated with large uncertainties and major knowledge gaps about DOC export pathways, mineralization and sequestration rates at both Nordic and global scales (Paine et al., 2021). Coarse estimates suggest that DOC may contribute more than 14% of the total C-sequestration supported by seagrasses and up to 67% of the total C-sequestration supported by macroalgae (Krause-Jensen and Duarte, 2016; Duarte and Krause-Jensen, 2017; Frigstad et al., 2021 and references herein).
Another knowledge gap is the potential emission of greenhouse gases (GHG) from Nordic BC habitats. Natural GHG’s that might escape from Nordic BC habitats are methane (CH4) and nitrous oxide (N2O), in addition of carbon dioxide (CO2), which may reduce the net climate change mitigation capacity (Al-Haj and Fulweiler, 2020). For eelgrass, a recent study on Nordic eelgrass sediments showed only minor emissions of methane (Asplund et al., 2022), and a study from the east coast of the USA documented that restored eelgrass meadows still represent a net sink of CO2 (0.42 t CO2e ha−1 yr−1) after including methane emission from the meadows (ca. 10% of the sequestration, Oreska et al., 2020). For salt marshes, the tidal regime as well as the salinity are important variables affecting the potential fluxes of methane or nitrous oxide emission and, thereby, the net C-sequestration capacity of marshes (Al-Haj and Fulweiler, 2020; Arias-Ortiz et al., 2021). Non-tidal and brackish salt marshes, such as those in the inner Baltic Sea, are likely especially prone to methane or nitrous oxide emission.
In addition to their C-sequestration capacity, marine vegetated habitats support a wide range of ecosystem functions and services such as (1) provisioning of biodiversity, including habitat and nursery grounds for economically important fish (birds in salt marshes), (2) a coastal nutrient filter that mitigates eutrophication, and (3) climate change buffering by natural coastal protection, alleviation of ocean acidification, and sediment accretion, (4) oxygen production, (5) protection of cultural heritage, and (6) aesthetical values. These aspects have received significant attention internationally (e.g. Costanza et al., 2014; Nordlund et al., 2016; Ruiz-Frau et al., 2017; Narayan et al., 2017) and will not be dealt with in any detail here. We solely underline that such functions are also documented in Nordic BC habitats (Table S6) and support the win-win aspect of the protection and restoration of BC habitats also in the Nordic region.
Management of Nordic Blue Carbon Habitats
This section gives an overview of the management of Nordic BC habitats in relation to protection against stressors, linking to the outlined policies, and with examples of concrete restoration initiatives. Most management addresses the BC habitats as such or BC sinks beyond the habitats both of which affect the capacity for climate change mitigation and adaptation as well as co-benefits of BC habitats.
Protection
Stressors differ somewhat between BC habitat types but include eutrophication and shading from coastal darkening, land-use changes/coastal development, physical disturbance from fisheries, overfishing, trawling, dredging, dumping, anchoring and harvest of macroalgae, as well as climate change (e.g.,Baden et al., 2010; Baden et al., 2012; Boström et al., 2014; Reusch et al., 2018; Frigstad et al., 2020; Krause-Jensen et al., 2021). Protective measures involve nutrient management plans, establishment of marine protected areas (MPAs) involving various controls on fisheries, dumping and anchoring in BC habitats and in C-sink areas beyond the BC habitats (e.g., Legge et al., 2020; Luisetti et al., 2020). Protection also involves controls on harvest of macroalgae as well as harvest/grazing as part of the protection of saltmarshes.
Nutrient Management
Plans to reduce eutrophication are widely implemented across the Nordic region, e.g. in response to the European directives (WFD, 2000; MFSD, 2008) and the HELCOM Baltic Sea Action Plan (https://helcom.fi/baltic-sea-action-plan/nutrient-reduction-scheme/targets/). In Denmark, for example, a first national Action Plan for the Aquatic Environment was enacted in 1987, followed by additional ones to achieve an overall objective of reducing total nitrogen and total phosphorus discharges by 50 and 80%, respectively. However, because 60% of the country is intensely cultivated, nutrient loadings remain a problem (Riemann et al., 2016).
Marine Protected Areas
MPAs in the Nordic region include sites designated under national laws as well as under the Natura 2000 network of nature protection areas. The latter encompasses Special Areas of Conservation under the Habitats Directive and Special Protection Areas under the Birds Directive (Figure 2C). Four of the Nordic MPAs are appointed UNESCO Marine World Heritage Sites: Surtsey (Iceland), West Norwegian Fjords – Geirangerfjord and Nærøyfjord (Norway), Wadden Sea (Germany/Netherlands/Denmark) and High Coast/Kvarken Archipelago (Finland) of which the first three support documented BC habitats (UNESCO, 2020).
The current proportion (in %) of “protected” marine area varies across the Nordic region with 4.5% in Norway [Meld. St. 29 (2020–2021) - regjeringen.no], 9.8% in Finland, 12.8% in Sweden and 15.2% in Denmark (https://biodiversity.europa.eu/countries/). However, the 2021 Nordic ministerial declaration on biodiversity, oceans and climate, the EU biodiversity strategy, the first draft of the CBD post-2020 global biodiversity and the High-level panel for a Sustainable Ocean Economy all support regional and global goals of 30% MPAs.
The actual protection of habitats within MPAs also varies considerably as the term “protection” is subject to interpretation. For the Baltic Sea in general, trawling remains a major stressor despite various controls (de Liedekere et al., 2020). In Denmark, fisheries have been allowed in most protected areas except in the Sound (Øresund) and except for a zone of 200 m surrounding stone reefs and bubble reefs, which implies that only about 2% of the marine areas are protected from trawling; however, a new marine plan aims to increase the targets. In NW Sweden, ~7% of the eelgrass area is negatively affected by small-scale construction of docks and marinas, even though habitats located less than 100 m from the shoreline are protected against exploitation by national law, and ~50% of the eelgrass area is located within protected areas (Eriander et al., 2017).
Under the Habitats Directive, salt marshes are designated a habitat type and are therefore more directly protected than eelgrass meadows and kelp forests, which just form part of the habitat types “Large shallow inlets and bays”, “estuaries” or “mudflats”. The Finnish Nature Conservation Act hence protects coastal meadows but not (yet) eelgrass meadows. In Iceland, national laws specify protection of mudflats and, thereby, eelgrass and salt marshes.
Controls on Harvest of Marine Vegetation
The harvest of wild kelp (Laminaria hyperborea) in Norway amounts to ~150,000 metric tons per year (Steen, 2018), representing ~0.3% of the estimated standing stock of living biomass (55 million metric tons, Frigstad et al., 2021; Gundersen et al., 2021). Usually, the Norwegian harvest area is ~6% of a regional kelp resource (Norderhaug et al., 2020), but can exceed 75% at monitoring points (Steen et al., 2018). The harvested yield has been stable for the past 30 years but is foreseen to increase as the number of commercial actors have recently doubled (from 1 to 2). Kelp harvest is regulated by law and regulations, and the Norwegian coast is divided into harvesting zones by latitude minutes (1 nautical mile), where each zone is open for harvest every 5-6 years, to allow for kelp regrowth. There is no upper limit on biomass harvest by regulations, and each zone can theoretically be depleted every 5 years. In practice, commercial actors prioritize areas with a high biomass per area and bottom conditions that fit the harvesting equipment. This often results in a patchy harvest. Harvest may be restricted based on the outcome of monitoring conducted half a year prior to planned harvesting. If the commercial demand is increasing in the future, and more efficient harvesting equipment is developed, macroalgae biomass, habitats and ecosystems may be severely impacted without further legislation.
The rockweed Ascophyllum nodosum is also harvested commercially in Norway, with yearly landings around 20,000 metric tons (Directorate of Fisheries, Norway, www.fiskeridir.no/tall-og-analyse/aapne-data). The Marine Resource Act does not regulate this harvesting, as it largely takes place in the intertidal, which is private property, and can be conducted with permission from the landowners.
In Iceland, the harvest of macroalgae is mainly confined to Breiðafjörður. The harvest of A. nodosum amounts to 15,000-20,000 metric tons per year and 4,000-7,000 metric tons for L. digitata and L. hyperborea (Ingólfsson, 2010; Gunnarsson et al., 2019). The Marine & Freshwater Research Institute has advised that the annual harvest 2018-2022 of A. nodosum in Breiðafjörður should not exceed 40,000 metric tons, or around 3% annually of estimated rockweed biomass in the area (Hafro.is, 2018).
There is limited tradition for commercial exploitation of wild macroalgae in Denmark, as well as in the Baltic Sea in general, but a significant harvest of pristine communities of the drifting red algae Furcellaria lumbricalis took place in the central Kattegat in the 1940-1960s and decimated the population (Schramm, 1998; Weinberger et al., 2020).
For salt marshes, overgrowth and shadowing by tall grass (e.g. reeds) is being managed by grazing and hay harvest to increase biodiversity. In Denmark, around two thirds of the total area of mapped salt marshes (2016-2019) is managed by such practices. In Finland, abandonment of traditional agricultural activities (Lehikoinen et al., 2017) has rendered boreal coastal meadows endangered (Schulman et al., 2008), and the conservation status under the EU Habitats Directive was assessed as unfavorable or bad in 2007 and 2013. However, due to new management efforts, the total area of Finnish salt marshes has now increased from 60 to 62 km2, and further supporting initiatives are in place via national funding (strategic nature conservation, restoration and management programme HELMI, 2020–2030) and EU funding (LIFE-project CoastNet LIFE, 2018-2025).
Restoration
The Nordic region holds several examples of active restoration of salt marshes, eelgrass meadows and kelp forests. Restoration can supplement protective measures and facilitate or speed up the natural recovery process. Below, we briefly summarize restoration techniques and provide (non-exhaustive) examples of restoration initiatives in the region (Figure 2D and Table S7). The Nordic restoration examples are typically individual projects that are not part of a coordinated large-scale effort and there is, as yet, no coordinated follow-up on restoration success, planning and guidance at national or Nordic scale.
Salt Marsh Restoration
As many former salt marsh areas were transformed to agriculture land by dikes, drainage and pumping, removal of these structures to reestablish the natural hydrology forms direct restoration measures. Such restoration links to “managed coastal realignment”, which is a new coastal protection strategy integrating coastal and nature protection by removing or abandoning coastal protection measures to reestablish natural processes and dynamics (Schernewski et al., 2018). There are examples from e.g. the southern Baltic Sea (Germany) and Denmark (Tryggelev Nor) (Karnauskaitė et al., 2018) citing the database “Our Coast” and the European Maritime Spatial Planning (MSP) platform database (www.msp-platform.eu). However, as saltmarshes are not necessarily positively conceived by the public, and engineering solutions to coastal protection are probably more generally acknowledged, there is a need for thorough public communication and involvement to build confidence and develop best practices (Stewart-Sinclair et al., 2020).
Eelgrass Restoration
Over the past decade, several eelgrass restoration efforts have been undertaken in the Nordic region (Figure 2D and Table S7). The activities have benefitted from international experience, which highlights (1) the need for careful site selection so that restoration takes place where eelgrass eelgrass used to grow and where habitat requirements are presently fulfilled, (2) the importance of sufficient spatial scale of the restoration to increase the chance of building resilience in the new patches, and (3) ensuring sufficient time perspective (years) for monitoring the effects of the restoration (Bayraktarov et al., 2016; van Katwijk et al., 2016; Orth et al., 2020). Persistent Nordic restoration efforts confirm these recommendations and provide best practices for site selection and full-scale restoration (e.g. Moksnes et al., 2016; Lange et al., 2022). The most successful Nordic eelgrass restorations have involved eelgrass transplants rather than seeds, and have sometimes involved anchoring of shoots, stabilization of sediments by protective structures, sandcapping, enclosures to avoid bioturbation, traps to reduce crabs, or co-restoration with blue mussels in order to facilitate eelgrass survival and growth (Moksnes et al., 2016; Gagnon et al., 2021; van der Heide et al., 2021, Flindt et al., 2022; Lange et al., 2022) (Table S7). Target areas for restoration have been identified by modelling where eelgrass habitat requirements are fulfilled (e.g. Canal-Vergés et al., 2016; Flindt et al., 2016) supplemented with further site inspection and transplantation trials (Lange et al., 2022). However, because eelgrass restoration is labor intensive, costly and with no guarantee of success, protection of existing meadows is a management priority (Moksnes et al., 2016).
Macroalgal Restoration
The main threats to macroalgae include but are not limited to removal of anchoring stones, aggressive fishery practices, and destructive grazing by sea urchins. In the cases of macroalgal habitat loss due to removal of stones, restoration of the habitat requires the establishment of new reefs. The Blue Reef project in the Kattegat (www.bluereef.dk) represents a successful example of macroalgal restoration. The experience gained from this and other projects led to a manual on best practices (Dahl et al., 2016a) and has inspired additional projects in Danish coastal waters (e.g. in Als Fjord and Limfjorden, and planned projects).
Restoration of macroalgae on barren grounds after destructive grazing by sea urchins has been carried out along the Norwegian coast by removing sea urchins, transplanting Laminaria hyperborea and Saccharina latissima kelp, planning sites of action taking interactions with other species, such as crabs, into account (Christie et al., 2019 and references therein). Based on this work, a strategy on how to recover kelp ecosystems from urchin barrens has been developed (Verbeek et al., 2021). “Green gravel” (i.e. small rocks seeded with kelp) has also recently been applied to restore sugar kelp (Fredriksen et al., 2020). The project MERCES has restored macroalgal habitats across Europe and concluded that active intervention (such as sea urchin removal) is required if the cause behind the habitat degradation and loss is not dealt with at a broader overall scale (Bekkby et al., 2020), e.g. by ensuring that intact fish populations control the sea urchin populations (Norderhaug et al., 2021). Internationally, Japan has the longest experience on macroalgal restoration including transplantation of kelp and removal of sea urchins, and their practice with country-wide restoration teams including fishermen (Watanuki et al., 2010; see also annex of Duarte et al., 2020) may inspire similar action in the Nordic region.
Macroalgal farming could potentially benefit wild macroalgal forests by leading to reduced harvest of wild macroalgae. Moreover, sustainable macroalgal farming may contribute associated ecosystem services, including uptake of excess nutrients, and supporting C-sequestration and reduced emissions, provided that the farming follows best practice sustainability standards (Duarte et al., 2022). Sustainability standards are needed to avoid potential negative effects of the farming such as competition of resources or physically damaging native habitats, and/or spreading of non-native strains (Campbell et al., 2019). Verified standards for C-accounting and C-offsetting connected with seaweed farming are also lacking and would require challenging and careful documentation (Hurd et al., 2022). The interest in the exploitation of macroalgae through harvest of living biomass and beach cast as well as via seaweed farming is on the rise both in the Nordic Atlantic region and the Baltic region, although the low salinity of the Baltic Sea presents suboptimal growth conditions for most species (Weinberger et al., 2020; Araújo et al., 2021). Among the Nordic countries, Norway has the largest number of seaweed farms (>27) and a large potential for macroalgal cultivation both inshore and offshore (Broch et al., 2019). The Norwegian seaweed cultivation industry is predicted to reach 4 million metric tons annually by 2030 and 20 million metric tons annually by 2050 (Olafsen et al., 2012). Macroalgal cultivation is also taking place in Denmark (6 farms), Faroe Island (2 farms), Greenland, Iceland and Sweden (one farm in each area) (Araújo et al., 2021).
Knowledge Gaps in Nordic Blue Carbon Science and Management
Scientific BC knowledge gaps include incomplete information on habitat area, changes in area, C-stocks, and C-sequestration rates and C-fluxes/budgets in general, including emission of methane and nitrous oxide. Another important information gap is to what extent it is possible to increase the C-sequestration of Nordic BC habitats via restoration and via protection against further loss, and thereby contribute to climate change mitigation. This is particularly the case for macroalgae for which information is lacking in each of the listed components, which prevents inclusion of macroalgal restoration and protection in BC policies. Salt marshes are also largely overlooked in BC context with very limited information on C-stocks and sequestration in the Nordic context, although areas are being assessed according to requirements of the Habitats Directive where this is relevant. The degree of success of restoration projects and their resulting ecosystem effects on C-sequestration are also poorly quantified as are large-scale ecosystem effects of macroalgal farming. Moreover, the effects of climate change on BC habitats need further exploration, both regarding plant communities, their performance and vulnerability, and regarding BC stocks and decomposition rates. These gaps limit both the possibility to quantify the climate change mitigation potential of Nordic BC habitats and their role in marine C-budgets at the Nordic scale. However, the recently completed Nordic Blue Carbon project provided, as mentioned, a major step forward in this direction for eelgrass and macroalgal communities (Frigstad et al., 2021). A recently initiated Nordic collaboration on salt marshes (“NordSalt” funded by EU) will increase the knowledge on Nordic salt marsh distribution, dynamics, C-storage, and management.
Managerial gaps include lacking a Nordic BC managerial roadmap, poor/unclear protection status of BC habitats, and limited focus on integration of management across sectors (e.g. environment/fisheries/climate/food), including optimizing management measures in the face of climate change. Regarding effects of climate change on the habitats, there is a need for considering projected changes in habitat extent and species ranges in relation to global change scenarios for the Nordic region. Perry et al. (2020) identified some areas in Sweden particularly vulnerable to combined global change effects, which should be considered in management schemes. Also, shallow Danish eelgrass meadows are more vulnerable to warming than deeper, cooler areas and management should facilitate the colonization of deeper areas by continued nutrient load reductions and prevention of mussel trawling (Krause-Jensen et al., 2021). Regarding potential effects of the habitats on the climate, there is only scattered knowledge on the mitigation potentials of Nordic BC habitats in terms of climate change mitigation and co-benefits (Gundersen et al., 2016; Frigstad et al., 2021).
Last but not least, further integration across sectors is needed, both science-management integration, integrated management of stressors to increase protection against eutrophication, physical damage as well as climate change, and integrated management of the functions of BC habitats in relation to biodiversity, climate change mitigation and adaptation, and nutrient filter effect.
A Nordic Blue Carbon Roadmap
The Nordic Blue Carbon project (nordicbluecarbon.no, Frigstad et al., 2021) recently highlighted policy recommendations, which we support and expand by also including salt marshes: “This group of scientists [.] urges for immediate and concerted policy actions to safeguard the Nordic Blue Carbon habitats, such as salt marshes, seagrass meadows, kelp forests and rockweed beds, especially through increased protection of coastal ecosystems by establishing marine protected areas and by increased efforts in reducing human pressures, such as nutrient pollution, overfishing and habitat fragmentation. There is enough scientific evidence to underpin the importance of these coastal ecosystems to support this call for action.” A similar message “Blue Carbon can’t wait” was also recently communicated as an editorial of the Science magazine (Douvere, 2021).
We propose a Nordic Blue Carbon roadmap for science and management along with a regional task force of Nordic experts to increase coordination and knowledge sharing, close the identified knowledge gaps, secure scientific data, and support the management of Nordic vegetated coastal and marine habitats as nature-based solutions to climate change with co-benefits for biodiversity and ecosystem health (Table 4). The science- and management roadmaps must be tightly connected, and linked to efficient communication to raise awareness to stimulate public and policy interest and involvement.
Among the key actions needed is to generate more evidence to understand and constrain the huge variability in C-storage and climate change mitigation potential of the Nordic BC habitats and understand how it varies along the steep environmental gradients. This involves better quantification of both areas and C-storage, as well as predictive models for the long-term C-storage capacity. Recent advances in remote sensing techniques (satellites and flying drones) facilitate large-scale spatial monitoring and enables mapping and monitoring of (at least the shallow) vegetation belts at Nordic scales, but further improvement is needed Future BC strategies should also identify the best methods and key target areas for restoration and protection based on historical distribution and habitat suitability in present and future climate scenarios, and have focus on habitat connectivity to guide. In addition, we recommend to explore the potential for including BC habitat in the Nordic countries’ NDCs or national greenhouse gas inventories. Importantly, such initiatives should supplement and not reduce efforts to cut fossil GHG emissions. While IPCC guidelines exist for seagrass and saltmarsh habitats, they are still missing for macroalgae (the brown algae being most relevant to the Nordic region), and a further action point is to support the development of macroalgal BC guidelines (Table 4).
The large attendance of both scientists, managers and policy makers to the Nordic BC meeting in Copenhagen in 2019 already then demonstrated the scope for a Nordic BC collaboration and the relevance of a Nordic BC roadmap (Table S8). The work discussed here shows that while progress is being made and there is recognition that more data collection and dissemination is needed, there remains key knowledge gaps and challenges to realizing the full climate benefit and co-benefits of Nordic BC systems. Blue Carbon is clearly a concept at the intersection of science and policy, stressing the need of good science to advise and guide policy that will lead to climate mitigation, adaptation through conservation and restoration of these ecosystems. A strategic roadmap, supported by a formalized Nordic BC platform that provides coordination, prioritization, and knowledge exchange etc., will be essential in generating future joint Nordic BC collaborations and solutions towards climate change mitigation.
Author Contributions
All authors contributed to the planning of the manuscript and provided information for their region and area of expertise. DK-J, HG and KH completed the first full draft, all authors approved the final version and JT also edited the references.
Funding
Velux Fonden provided support to DK-J, MH, AG, and GB throughthe project “Blå Skove (Blue Forests), no. 28421” and throughsupport for the BCI workshop in Copenhagen. The Copenhagenworkshop was also supported by NASA (grant number:80NSSC19K1627) to BCI. The Norwegian Environment Agencyand the Nordic Council of Ministers provided support via theNordic Blue Carbon project (“Blue Carbon – climate adaptation,CO2 uptake and sequestration of carbon in Nordic blue forests”;https://nordicbluecarbon.no/). DK-J also received funding fromthe Independent Research Fund Denmark (CARMA; grantnumber: 8021-00222B) regarding Greenland and from theEuropean Commission H2020 (FutureMARES; grant number:869300) regarding the Baltic Sea. CB received financial supportfrom the Åbo Akademi University Foundation Sr. We furtheracknowledge economic support from the Foundation for Balticand East European studies (Östersjöstiftelsen) via the projectClimScape led by MG (grant number: 21-GP-0005). KH, HG,TB, SS, and HF received funding from the Norwegian Institute forWater Research (NIVA).
Conflict of Interest
The authors declare that the research was conducted in the absence of any commercial or financial relationships that could be construed as a potential conflict of interest.
Publisher’s Note
All claims expressed in this article are solely those of the authors and do not necessarily represent those of their affiliated organizations, or those of the publisher, the editors and the reviewers. Any product that may be evaluated in this article, or claim that may be made by its manufacturer, is not guaranteed or endorsed by the publisher.
Acknowledgments
All participants at the Blue Carbon Initiative’s (BCI) Nordic Blue Carbon workshop, Copenhagen, September 2019 are thanked for their contributions. Ole Geertz-Hansen is, in particular, thanked for information on marine management in Greenland.
Supplementary Material
The Supplementary Material for this article can be found online at: https://www.frontiersin.org/articles/10.3389/fmars.2022.847544/full#supplementary-material
References
Adam P. (1990). Saltmarsh Ecology (Cambridge: Cambridge University Press). doi: 10.1017/CBO9780511565328
Al-Haj A. N., Fulweiler R. W. (2020). A Synthesis of Methane Emissions From Shallow Vegetated Coastal Ecosystems. Glob. Change Biol. 26, 2988–3005. doi: 10.1111/gcb.15046
Andersen J. H., Halpern B. S., Korpinen S., Murray C., Reker J. (2015). Baltic Sea Biodiversity Status vs. Cumulative Human Pressures. Estuar. Coast. Shelf Sci. 161, 88–92. doi: 10.1016/j.ecss.2015.05.002
Aoki L. R., McGlathery K. J., Oreska M. P. J. (2020). Seagrass Restoration Reestablishes the Coastal Nitrogen Filter Through Enhanced Burial. Limnol. Oceanogr. 65, 1–12. doi: 10.1002/lno.11241
Araújo R. M., Assis J., Aguillar R., Airoldi L., Bárbara I., Bartsch I., et al. (2016). Status, Trends and Drivers of Kelp Forests in Europe: An Expert Assessment. Biodivers. Conserv. 25, 1319–1348. doi: 10.1007/s10531-016-1141-7
Araújo R., Vázquez Calderón F., Sánchez López J., Azevedo I. C., Bruhn A., Fluch S., et al. (2021). Current Status of the Algae Production Industry in Europe: An Emerging Sector of the Blue Bioeconomy. Front. Mar. Sci. 7. doi: 10.3389/fmars.2020.626389
Arias-Ortiz A., Oikawa P. Y., Carlin J., Masqué P., Shahan J., Kanneg S., et al. (2021). Tidal and Nontidal Marsh Restoration: A Trade‐Off Between Carbon Sequestration, Methane Emissions, and Soil Accretion. J. Geophys. Res.Biogeosci. 126 (12), e2021JG006573.
Asplund M. E., Bonaglia S., Boström C., Dahl M., Deyanova D., Gagnon K., et al. (2022). Methane Emissions From Nordic Seagrass Meadow Sediments. Front. Mar. Sci. 8. doi: 10.3389/fmars.2021.811533
Baden S., Boström C., Tobiasson S., Arponen H., Moksnes P. O. (2010). Relative Importance of Trophic Interactions and Nutrient Enrichment in Seagrass Ecosystems: A Broad-Scale Field Experiment in the Baltic-Skagerrak Area. Limnol. Oceanogr. 55, 1435–1448. doi: 10.4319/lo.2010.55.3.1435
Baden S., Emanuelsson A., Pihl L., Svensson C. J., Åberg P. (2012). Shift in Seagrass Food Web Structure Over Decades is Linked to Overfishing. Mar. Ecol. Prog. Ser. 451, 61–73. doi: 10.3354/meps09585
Baden S., Gullström M., Lunden B., Pihl L., Rosenberg R. (2003). Vanishing Seagrass (Zostera Marina, L.) in Swedish Coastal Waters. Ambio 32, 374–377. doi: 10.1579/0044-7447-32.5.374
Barbier E. B., Hacker S. D., Kennedy C., Koch E. W., Stier A. C., Silliman B. R. (2011). The Value of Estuarine and Coastal Ecosystem Services. Ecol. Monogr. 81, 169–193. doi: 10.1890/10-1510.1
Bayraktarov E., Saunders M. I., Abdullah S., Mills M., Beher J., Possingham H. P., et al. (2016). The Cost and Feasibility of Marine Coastal Restoration. Ecol. Appl. 26, 1055–1074. doi: 10.1890/15-1077
Bekkby T., Moy F. E., Olsen H., Rinde E., Bodvin T., Bøe R., et al. (2013). “The Norwegian Programme for Mapping of Marine Habitats – Providing Knowledge and Maps for ICZMP,” in Global Challenges in Integrated Coastal Zone Management. Eds. Moksness E., Dahl E., Støttrup J.. Oxford, UK: John Wiley & Sons, Ltd, 19–30. doi: 10.1002/9781118496480.ch2
Bekkby T., Papadopoulou N., Fiorentino D., McOwen C. J., Rinde E., Boström C., et al. (2020). Habitat Features and Their Influence on the Restoration Potential of Marine Habitats in Europe. Front. Mar. Sci. 7. doi: 10.3389/fmars.2020.00184
Blomqvist M., Olsson P. (2007). Översyn Av Det Nationella Marina Övervakningsprogrammet För Vegetationsklädda Bottnar (Stockholm, Sweden: Swedish Environmental Protection Agency). Available at: http://www.diva-portal.se/smash/get/diva2:717044/FULLTEXT01.pdf.
Borgersen G., Rinde E., Moy S., Gundersen H. (2020) Are There Saltmarshes in Norway? An Assessment of the Term Against Norwegian Habitat Types (NiN). NIVA Report 7558-2020. Available at: https://hdl.handle.net/11250/2718857.
Boström C., Baden S., Bockelmann A. C., Dromph K., Fredriksen S., Gustafsson C., et al. (2014). Distribution, Structure and Function of Nordic Eelgrass (Zostera Marina) Ecosystems: Implications for Coastal Management and Conservation. Aquat. Conserv. 24, 410–434. doi: 10.1002/aqc.2424
Boström C., Baden S. P., Krause-Jensen D. (2003). “The Seagrasses of Scandinavia and the Baltic Sea,” in World Atlas of Seagrasses. Eds. Green E. P., Short F. T. (Berkeley, USA: University of California Press), 27–37.
Boström C., Bonsdorff E., Kangas P., Norkko A. (2002). Long-Term Changes of a Brackish-Water Eelgrass (Zostera Marina L.) Community Indicate Effects of Coastal Eutrophication. Estuar. Coast. Shelf Sci. 55, 795–804. doi: 10.1006/ecss.2001.0943
Boysen-Jensen P. (1914). Studies Concerning the Organic Matter of the Sea Bottom. Rep. Dan. Biol. Stat. 22, 1–39.
Broch O. J., Alver M. O., Bekkby T., Gundersen H., Forbord S., Handa A., et al. (2019). The Kelp Cultivation Potential in Coastal and Offshore Regions of Norway. Front. Mar. Sci. 5. doi: 10.3389/fmars.2018.00529
Bruntse G., Lein T. E., Nielsen R. (1999). Marine Benthic Algae and Invertebrate Communities From the Shallow Waters of the Faroe Islands - A Baseline Study (Tórshavn, The Faroe Islands: Kalbak Marine Biological Laboratory, the Faroe Islands).
Bültmann H., Daniëls F. J. (2013) Greenland Data Stored in the Arctic Vegetation Archive (AVA) in Münster. (Eds). Walker D. A., Breen A. L., Raynolds M. K., Walker M. D. In: Arctic Vegetation Archive (AVA) Workshop, Krakow, Poland, April 14-16, 2013. CAFF Proceedings Report 10 (Iceland: CAFF). Available at: www.researchgate.net/publication/257021400_Arctic_Vegetation_Archive_AVA_Workshop_Proceedings (Accessed 2021-12-15).
Campbell I., Macleod A., Sahlmann C., Neves L., Funderud J., Øverland M., et al. (2019). The Environmental Risks Associated With the Development of Seaweed Farming in Europe-Prioritizing Key Knowledge Gaps. Front. Mar. Sci. 6. doi: 10.3389/fmars.2019.00107
Canal-Vergés P., Petersen J. K., Rasmussen E. K., Erichsen A., Flindt M. R. (2016). Validating GIS Tool to Assess Eelgrass Potential Recovery in the Limfjorden (Denmark). Ecol. Model. 338, 135–148. doi: 10.1016/j.ecolmodel.2016.04.023
Christie H., Andersen G. S., Bekkby T., Fagerli C. W., Gitmark J. K., Gundersen H., et al. (2019). Shifts Between Sugar Kelp and Turf Algae in Norway: Regime Shifts or Fluctuations Between Different Opportunistic Seaweed Species? Front. Mar. Sci. 6. doi: 10.3389/fmars.2019.00072
Costanza R., de Groot R., Sutton P., van der Ploeg S., Anderson S. J., Kubiszewski I., et al. (2014). Changes in the Global Value of Ecosystem Services. Global Environ. Change 26, 152–158. doi: 10.1016/j.gloenvcha.2014.04.002
Dahl M., Asplund M. E., Björk M., Deyanova D., Infantes E., Isaeus M., et al. (2020a). The Influence of Hydrodynamic Exposure on Carbon Storage and Nutrient Retention in Eelgrass (Zostera Marina L.) Meadows on the Swedish Skagerrak Coast. Sci. Rep. 10, 13666. doi: 10.1038/s41598-020-70403-5
Dahl M., Asplund M. E., Deyanova D., Franco J. N., Koliji A., Infantes E., et al. (2020b). High Seasonal Variability in Sediment Carbon Stocks of Cold-Temperate Seagrass Meadows. J. Geophys. Res. Biogeosci. 125, e2019JG005430. doi: 10.1029/2019JG005430
Dahl M., Deyanova D., Gutschow S., Asplund M. E., Lyimo L. D., Karamfilov V., et al. (2016b). Sediment Properties as Important Predictors of Carbon Storage in Zostera Marina Meadows: A Comparison of Four European Areas. PLoS One 11, e0167493. doi: 10.1371/journal.pone.0167493
Dahl K., Lundsteen S., Helmig S. (2003). Stenrev, Havbundens Oaser (Copenhagen, Denmark: Gads Forlag). Available at: http://www2.dmu.dk/1_viden/2_Publikationer/3_miljobib/rapporter/MB02.pdf.
Dahl E., Naustvoll L. J., Steen H., Bodvin T. (2008) Utredning Om Bruk Av Ålegras Som Biologisk Kvalitetselement I Forbindelse Med Vannforskriften.Norwegian Pollution Control Authority Ta2464/2008. Available at: https://evalueringsportalen.no/evaluering/eu-s-rammedirektiv-for-vann-utredning-om-bruk-av-aalegress-til-klassifisering-av-okologisk-tilstand/ta2464.pdf/@@inline.
Dahl K., Støttrup J. G., Stenberg C., Berggreen U. C., Jensen J. H. (2016a). Best Practice for Restoration of Stone Reefs in Denmark (Codes of Conduct) (Aarhus, Denmark: Aarhus University, DCE – Danish Centre for Environment and Energy. Technical Report no. 91). Available at: http://dce2.au.dk/pub/TR91.pdf.
de Liedekerke V., Thoreson O., Owen S., Berggren H. G. (2020). A Sea Under Pressure: Bottom Trawling Impacts in the Baltic (Solna, Sweden: WWF-Baltic Ecoregion Programme). Available at: www.wwfbaltic.org/report/a-sea-under-pressure-bottom-trawling-impacts-in-the-baltic/.
Diesing M., Thorsnes T., Bjarnadottir L. R. (2021). Organic Carbon Densities and Accumulation Rates in Surface Sediments of the North Sea and Skagerrak. Biogeosciences 18, 2139–2160. doi: 10.5194/bg-18-2139-2021
Downie A. L., von Numers M., Boström C. (2013). Influence of Model Selection on the Predicted Distribution of the Seagrass Zostera Marina. Estuar Coast Shelf S 121, 8–19. doi: 10.1016/j.ecss.2012.12.020
Duarte C. M., Agusti S., Barbier E., Britten G. L., Castilla J. C., Gattuso J.-P., et al. (2020). Rebuilding Marine Life. Nature 580, 39–51. doi: 10.1038/s41586-020-2146-7
Duarte C. M., Bruhn A., Krause-Jensen D. (2022). A Seaweed Aquaculture Imperative to Meet Global Sustainability Targets. Nat. Sustainability 5, 185–193. doi: 10.1038/s41893-021-00773-9
Duarte C. M., Krause-Jensen D. (2017). Export From Seagrass Meadows Contributes to Marine Carbon Sequestration. Front. Mar. Sci. 4. doi: 10.3389/fmars.2017.00013
Duarte C. M., Losada I. J., Hendriks I. E., Mazarrasa I., Marbà N. (2013). The Role of Coastal Plant Communities for Climate Change Mitigation and Adaptation. Nat. Climate Change 3, 961–968. doi: 10.1038/nclimate1970
Dunic J. C., Brown C. J., Connolly R. M., Turschwell M. P., Côté I. M. (2021). Long-Term Declines and Recovery of Meadow Area Across the World’s Seagrass Bioregions. Glob. Change Biol. 27, 4096–4109. doi: 10.1111/gcb.15684
Envall M., Isaksson I. (2012). Ålgräsutbredning (Zostera Sp.) I Västra Götalands Län Sommaren 2008. Länsstyrelsen I Västra Götalands Län, Vattenvårdsenheten. Report No. 2012:58. Länsstyrelsen report series, Västra Götalands län, Sweden
Eriander L., Laas K., Bergström P., Gipperth L., Moksnes P. O. (2017). The Effects of Small-Scale Coastal Development on the Eelgrass (Zostera Marina L.) Distribution Along the Swedish West Coast - Ecological Impact and Legal Challenges. Ocean Coast. Manage. 148, 182–194. doi: 10.1016/j.ocecoaman.2017.08.005
Espeland S. H., Knutsen H. (2014). Rapport fra høstundersøkelsene med strandnot i Indre Oslofjord 2014. Havforskningsinstituttet 31-2014, 1–15.
Evans D., Roekarts M. (2019). Interpretation Manual of the Habitats Listed in Resolution No. 4 (1996) Listing Endangered Natural Habitats Requiring Specific Conservation Measures. Fourth Draft Version 2019 (Council of Europe, Strasbourg). Available at: https://rm.coe.int/interpretation-manual-of-the-habitats-listed-in-resolution-no-4-1996-/168098c68c
Filbee-Dexter K., Wernberg T. (2018). Rise of Turfs: A New Battlefront for Globally Declining Kelp Forests. Bioscience 68, 64–76. doi: 10.1093/biosci/bix147
Filbee-Dexter K., Wernberg T., Grace S. P., Thormar J., Fredriksen S., Narvaez C. N., et al. (2020). Marine Heatwaves and the Collapse of Marginal North Atlantic Kelp Forests. Sci. Rep. 10, 13388. doi: 10.1038/s41598-020-70273-x
Filbee-Dexter K., Wernberg T., Norderhaug K. M., Ramirez-Llodra E., Pedersen M. F. (2018). Movement of Pulsed Resource Subsidies From Kelp Forests to Deep Fjords. Oecologia 187, 291–304. doi: 10.1007/s00442-018-4121-7
Flindt M. R., Oncken N. S., Kuusemäe K., Lange T., Aaskoven N., Winter S., et al. (2022). Sand-Capping Stabilizes Muddy Sediment and Improves Benthic Light Conditions in Eutrophic Estuaries: Laboratory Verification and the Potential for Recovery of Eelgrass (Zostera Marina). J. Sea Res. 181, 102177. doi: 10.1016/j.seares.2022.102177
Flindt M. R., Rasmussen E. K., Valdemarsen T., Erichsen A., Kaas H., Canal-Vergés P. (2016). Using a GIS-Tool to Evaluate Potential Eelgrass Reestablishment in Estuaries. Ecol. Model. 338, 122–134. doi: 10.1016/j.ecolmodel.2016.07.005
Fredriksen S., Christie H., Saethre B. A. (2005). Species Richness in Macroalgae and Macrofauna Assemblages on Fucus Serratus L. (Phaeophyceae) and Zostera Marina L. (Angiospermae) in Skagerrak, Norway. Mar. Biol. Res. 1, 2–19. doi: 10.1080/17451000510018953
Fredriksen S., Filbee-Dexter K., Norderhaug K. M., Steen H., Bodvin T., Coleman M. A., et al. (2020). Green Gravel: A Novel Restoration Tool to Combat Kelp Forest Decline. Sci. Rep. 10, 3983. doi: 10.1038/s41598-020-60553-x
Fredshavn J., Nygaard B., Ejrnæs R., Damgaard C., Therkildsen O. R., Elmeros M., et al. (2019) Bevaringsstatus for Naturtyper Og Arter - 2019. Habitatdirektivets Artikel 17-Rapportering. Aarhus Universitet, DCE - Nationalt Center for Miljø Og Energi. Scientific Report No. 340. Available at: http://dce2.au.dk/pub/SR340.pdf.
Frigstad H., Andersen G. S., Trannum H. C., Naustvoll L. J., Kaste Ø., Hjermann D.Ø. (2018) Synthesis of Climate Relevant Results From Selected Monitoring Programs in the Coastal Zone. Part 2: Quantitative Analyses. Norwegian Institute for Water Research. NIVA Report 7311-2018. Available at: http://hdl.handle.net/11250/2595792.
Frigstad H., Gundersen H., Andersen G. S., Borgersen G., Kvile K.Ø., Krause-Jensen D., et al. (2021). “Blue Carbon – Climate Adaptation, CO2 Uptake and Sequestration of Carbon in Nordic Blue Forests – Results From the Nordic Blue Carbon Project,” (Copenhagen: Nordic Council of Ministers), TemaNord2020: 541. doi: 10.6027/temanord2020-541
Frigstad H., Harvey T., Deininger A., Poste A. (2020). Increased Light Attenuation in Norwegian Coastal Waters – a Literature Review. NIVA-Report 7551-2020 (Grimstad: Norwegian Institute for Water Research). Available at: https://hdl.handle.net/11250/2711599.
Gagnon K., Christie H., Didderen K., Fagerli C. W., Govers L. L., Gräfnings M. L. E., et al. (2021). Incorporating Facilitative Interactions Into Small-Scale Eelgrass Restoration-Challenges and Opportunities. Restor. Ecol. 29, e13398. doi: 10.1111/rec.13398
Gattuso J.-P., Magnan A. K., Bopp L., Cheung W. W. L., Duarte C. M., Hinkel J., et al. (2018). Ocean Solutions to Address Climate Change and Its Effects on Marine Ecosystems. Front. Mar. Sci. 5. doi: 10.3389/fmars.2018.00337
Glooschenko W. A., Tarnocai C., Zoltai S., Glooschenko V. (1993). “Wetlands of Canada and Greenland,” in Wetlands of the World: Inventory, Ecology and Management, vol. I . Eds. Whigham D. F., Dykyjová D., Hejný S. (Dordrecht: Springer Netherlands), 415–514. doi: 10.1007/978-94-015-8212-4
Graversen A. E. G., Banta G. T., Masque P., Krause-Jensen D. (2022). Carbon Sequestration is Not Inhibited by Livestock Grazing in Danish Salt Marshes. Limnol. Oceanogr. doi: 10.1002/(ISSN)1939-5590
Gudbrandsson G. I., Wermelin L., Mikkonen K., Elvestuen O., Lövin I., Gunell C., et al. (2019) “Nordic Ministerial Declaration on Oceans and Climate – for adoption at the Nordic Ministerial Meeting in Stockholm, 30 October 2019” (Stockholm: Nordic Council of Ministers for the Environment and Climate). Available at: www.norden.org/en/declaration/nordic-ministerial-declaration-oceans-andclimate.
Gullström M., Isæus M., Berglund J., Blomqvist M., Karlsson A., Nygård L., et al. (2009). Övervakning Av Makrovegetation I Bottniska Viken – En Vägledning (in English: Monitoring of Macrovegetation in Bothnian Bay – a Guide) (Meddelande: Länsstyrelsens Västerbotten), 6. Available at: http://naturvardsverket.diva-portal.org/smash/record.jsf?pid=diva2:770191.
Gundersen H., Bekkby T., Oug E., Norderhaug K. M., Fredriksen S., Rinde E. (2018). Marine Shallow Waters. Norwegian Redlist for Habitats 2018 (Trondheim: Norwegian Biodiversity Information Centre). Available at: www.artsdatabanken.no/Pages/259183.
Gundersen H., Bryan T., Chen W., Moy F. E. (2016). Ecosystem Services. In The Coastal Zone of the Nordic Countries 552 (Copenhagen: Nordic Council of Ministers), 127. doi: 10.6027/TN2016-552
Gundersen H., Rinde E., Bekkby T., Hancke K., Gitmark J. K., Christie H. (2021). Variation in Population Structure and Standing Stocks of Kelp Along Multiple Environmental Gradients and Implications for Ecosystem Services. Front. Mar. Sci. 8. doi: 10.3389/fmars.2021.578629
Gunnarsson K., Burgos J., Gunnarsdóttir L., Egilsdóttir S., Georgsdóttir G. I., Madrigal V. F. P. (2019). Klóþang Í Breiðafirði: Útbreiðsla Og Magn. Hafrannsóknastofnun HV 2019-16. Available at: www.hafogvatn.is/static/research/files/hv2019-16.pdf.
Hammar L., Schmidtbauer Crona J., Kågesten G., Hume D., Pålsson J,, Aarsrud M., et al (2018). Symphony: Integrerat planeringsstöd för statlig havsplanering utifrån en ekosystemansats. (Swedish: Swedish Agency for Marine and Water Management report) 2018:1. Available at: http://urn.kb.se/resolve?urn=urn:nbn:se:havochvatten:diva-170
Høgslund S., Sejr M. K., Wiktor J., Blicher M. E., Wegeberg S. (2014). Intertidal Community Composition Along Rocky Shores in South-West Greenland: A Quantitative Approach. Polar Biol. 37, 1549–1561. doi: 10.1007/s00300-014-1541-7
Hafro.is (2018) State of Marine Stocks and Advise 2018 - KlóÞang – Rockweed. Available at: www.hafogvatn.is/static/extras/images/thang2018318234.pdf.
Halvorsen R., Skarpaas O., Bryn A., Bratli H., Erikstad L., Simensen T., et al. (2020). Towards a Systematics of Ecodiversity: The EcoSyst Framework. Global Ecol. Biogeogr. 29, 1887–1906. doi: 10.1111/geb.13164
HELCOM (2013). ’HELCOM HUB – Technical Report on the HELCOM Underwater Biotope and Habitat Classification,’ in Baltic Sea Environment Proceedings 139. Helsinki: HELCOM. Available at: www.helcom.fi/Lists/Publications/BSEP139.pdf.
Hoegh-Guldberg O., Caldeira K., Chopin T., Gaines S., Haugan P., Hemer M., et al. (2019). The Ocean as a Solution to Climate Change: Five Opportunities for Action. Report (Washington, DC: World Resources Institute). Available at: http://www.oceanpanel.org/climate.
Hurd C. L., Law C. S., Bach L. T., Britton D., Hovenden M., Paine E., et al (2022). Forensic Carbon Accounting: Assessing the Role of Seaweeds for Carbon Sequestration. J. Phycol. doi: 10.1111/jpy.13249
Ingólfsson A. (2010). Náttúruverndargildi Íslensku Fjörunnar Og Aðsteðjandi Hættur. Náttúrufræðingurinn 79, 19–28.
IPCC (2014). 2013 Supplement to the 2006 IPCC Guidelines for National Greenhouse Gas Inventories: Wetlands. Eds. Hiraishi T., Krug T., Tanabe K., Srivastava N., Baasansuren J., Fukuda M., Troxler T. G. (Switzerland: IPCC).
Jakobsen B. (1954). The Tidal Area in South-Western Jutland and the Process of the Salt Marsh Formation. Geografisk Tidsskrift 53, 49–61.
Janssen J. A. M., Rodwell J. S. (2016). European Red List of Habitats : Part 2. Terrestrial and Freshwater Habitats (Luxembourg: Publications Office of the European Union). doi: 10.2779/091372
Jensen L. A., Schmidt L. B., Hollesen J., Elberling B. (2006). Accumulation of Soil Organic Carbon Linked to Holocene Sea Level Changes in West Greenland. Arct. Antarct. Alp. Res. 38, 378–383. doi: 10.1657/1523-0430(2006)38[378:AOSOCL]2.0.CO;2
Jóhansen J., Fosaa A. M., Rasmussen S. (Eds.) (2000). Føroysk Flora (Føroya skúlabókagrunnur: Tórshavn).
Karlsson J. (2007). Övervakning Av Vegetationsklädda Hårdbottnar Vid Svenska Västkusten 1993-2006 (Gothenburg, Sweden: Tjärnö marinbiologiska laboratorium, Göteborgs Universitet). Available at: http://naturvardsverket.diva-portal.org/smash/record.jsf?pid=diva2:657799.
Karnauskaitė D., Schernewski G., Schumacher J., Grunert R., Povilanskas R. (2018). Assessing Coastal Management Case Studies Around Europe Using an Indicator Based Tool. J. Coast. Conserv 22, 549–570. doi: 10.1007/s11852-018-0597-x
Kautsky N., Kautsky H., Kautsky U., Waern M. (1986). Decreased Depth Penetration of Fucus Vesicolosus (L.) Since the 1940's Indicates Eutrophication of the Baltic Sea. Mar. Ecol. Prog. Ser. 28, 1–8. doi: 10.3354/meps028001
Kindeberg T., Ørberg S. B., Röhr M. E., Holmer M., Krause-Jensen D. (2018). Sediment Stocks of Carbon, Nitrogen, and Phosphorus in Danish Eelgrass Meadows. Front. Mar. Sci. 5. doi: 10.3389/fmars.2018.00474
Krause-Jensen D., Archambault P., Assis J., Bartsch I., Bischof K., Filbee-Dexter K., et al. (2020). Imprint of Climate Change on Pan-Arctic Marine Vegetation. Front. Mar. Sci. 7. doi: 10.3389/fmars.2020.617324
Krause-Jensen D., Duarte C. M. (2016). Substantial Role of Macroalgae in Marine Carbon Sequestration. Nat. Geosci 9, 737–742. doi: 10.1038/Ngeo2790
Krause-Jensen D., Duarte C. M., Sand-Jensen K., Carstensen J. (2021). Century-Long Records Reveal Shifting Challenges to Seagrass Recovery. Glob. Change Biol. 27, 563–575. doi: 10.1111/gcb.15440
Krause-Jensen D., Marbà N., Olesen B., Sejr M. K., Christensen P. B., Rodrigues J., et al. (2012). Seasonal Sea Ice Cover as Principal Driver of Spatial and Temporal Variation in Depth Extension and Annual Production of Kelp in Greenland. Glob. Change Biol. 18, 2981–2994. doi: 10.1111/j.1365-2486.2012.02765.x
Krause-Jensen D., Lavery P., Serrano O., Marbà N., Masque P., Duarte C. M. (2018). Sequestration of Macroalgal Carbon: The Elephant in the Blue Carbon Room. Biol. Letters 14 (6), 20180236. doi: 10.1098/rsbl.2018.0236
Krause-Jensen D., Sejr M. K., Bruhn A., Rasmussen M. B., Christensen P. B., Hansen J. L. S., et al. (2019). Deep Penetration of Kelps Offshore Along the West Coast of Greenland. Front. Mar. Sci. 6. doi: 10.3389/fmars.2019.00375
Lange T., Oncken N. S., Svane N., Steinfurth R. C., Kristensen E., Flindt M. R. (2022). Large-Scale Eelgrass Transplantation: A Measure for Carbon and Nutrient Sequestration in Estuaries. Marine Ecol. Prog. Ser. 685, 97–109. doi: 10.3354/meps13975
Legge O., Johnson M., Hicks N., Jickells T., Diesing M., Aldridge J., et al. (2020). Carbon on the Northwest European Shelf: Contemporary Budget and Future Influences. Front. Mar. Sci. 7. doi: 10.3389/fmars.2020.00143
Lehikoinen P., Lehikoinen A., Mikkola-Roos M., Jaatinen K. (2017). Counteracting Wetland Overgrowth Increases Breeding and Staging Bird Abundances. Sci. Rep. 7, 41391. doi: 10.1038/srep41391
Lehtomaa L., Ahonen I., Hakamäki H., Häggblom M., Jantunen J., Jutila H., et al. (2018). “Perinnebiotoopit. [Traditional Rural Biotopes],” in Suomen Luontotyyppien Uhanalaisuus 2018. Luontotyyppien Punainen Kirja – Osa 2: Luontotyyppien Kuvaukset. (Threatened Habitat Types in Finland 2018. Red List of Habitats – Part II: Descriptions of Habitat Types). Eds. Kontula T., Raunio A. (Finnish Environment Institute and Ministry of the Environment. Finnish Environment), 659–757.
Lepping O., Daniels F. J. A. (2007). Phytosociology of Beach and Salt Marsh Vegetation in Northern West Greenland. Polarforschung 76, 95–108. doi: 10013/epic.29958.d001
Lindegarth M., Carstensen J., Drakare S., Johnson R. K., Sandman A. N., Söderpalm A., et al. (2016). Ecological Assessment of Swedish Water Bodies; Development, Harmonisation and Integration of Biological Indicators. Final Report of the Research Programme WATERS. Deliverable 1.1-4. WATERS Report No. 2016 (Sweden: Havsmiljöinstitutet), 10.
Long A. J., Woodroffe S. A., Milne G. A., Bryant C. L., Simpson M. J. R., Wake L. M. (2012). Relative Sea-Level Change in Greenland During the Last 700 Yrs and Ice Sheet Response to the Little Ice Age. Earth Planet Sci. Lett. 315, 76–85. doi: 10.1016/j.epsl.2011.06.027
Lovelock C. E., Atwood T., Baldock J., Duarte C. M., Hickey S., Lavery P. S., et al. (2017). Assessing the Risk of Carbon Dioxide Emissions From Blue Carbon Ecosystems. Front. Ecol. Environ. 15, 257–265. doi: 10.1002/fee.1491
Luisetti T., Ferrini S., Grilli G., Jickells T. D., Kennedy H., Kröger S., et al. (2020). Climate Action Requires New Accounting Guidance and Governance Frameworks to Manage Carbon in Shelf Seas. Nat. Commun. 11, 4599. doi: 10.1038/s41467-020-18242-w
Lundén B., Gullström M. (2013). Satellite Remote Sensing for Monitoring of Vanishing Seagrass in Swedish Coastal Waters. Nor. Geogr. Tidsskr. 57, 121–124. doi: 10.1080/00291950310001379
Macreadie P. I., Costa M. D. P., Atwood T. B., Friess D. A., Kelleway J. J., Kennedy H., et al. (2021). Blue Carbon as a Natural Climate Solution. Nat. Rev. Earth Env. 2, 826–839. doi: 10.1038/s43017-021-00224-1
Marbà N., Krause-Jensen D., Masque P., Duarte C. M. (2018). Expanding Greenland Seagrass Meadows Contribute New Sediment Carbon Sinks. Sci. Rep. 8, 14024. doi: 10.1038/s41598-018-32249-w
Mcleod E., Chmura G. L., Bouillon S., Salm R., Björk M., Duarte C. M., et al. (2011). A Blueprint for Blue Carbon: Toward an Improved Understanding of the Role of Vegetated Coastal Habitats in Sequestering CO2. Front. Ecol. Environ. 9, 552–560. doi: 10.1890/110004
Middelboe A. L., Sand-Jensen K., Brodersen K. (1997). Patterns of Macroalgal Distribution in the Kattegat-Baltic Region. Phycologia 36, 208–219. doi: 10.2216/i0031-8884-36-3-208.1
Moksnes P.-O., Gipperth L., Eriander L., Laas K., Cole S., Infantes E. (2016). Handbok För Restaurering Av Ålgräs I Sverige – Vägledning. Havs Och Vattenmyndigheten. Report No. 2016. 9, Vattenmyndigheten (the Swedish Agency for Marine and Water Management), Göteborg, Sweden. Available at: http://urn.kb.se/resolve?urn=urn:nbn:se:havochvatten:diva-108 Vattenmyndigheten (the Swedish Agency for Marine and Water Management), Göteborg, Sweden, Available from www.researchgate.net/publication/311512377_Handbook_for_eelgrass_restoration_in_Sweden_-_A_guideline.
Moksnes P. O., Gullström M., Tryman K., Baden S. (2008). Trophic Cascades in a Temperate Seagrass Community. Oikos 117, 763–777. doi: 10.1111/j.0030-1299.2008.16521.x
Moksnes P. O., Röhr M. E., Holmer M., Eklöf J. S., Eriander L., Infantes E., et al. (2021). Major Impacts and Societal Costs of Seagrass Loss on Sediment Carbon and Nitrogen Stocks. Ecosphere 12, e03658. doi: 10.1002/ecs2.3658
MSFD (2008). Directive 2008/56/EC of the European Parliament and of the Council of 17 June 2008 Establishing a Framework for Community Action in the Field of Marine Environmental Policy (Marine Strategy Framework Directive. Official Journal of the European Union).
Mueller P., Ladiges N., Jack A., Schmiedl G., Kutzbach L., Jensen K., et al. (2019). Assessing the Long-Term Carbon-Sequestration Potential of the Semi-Natural Salt Marshes in the European Wadden Sea. Ecosphere 10, e02556. doi: 10.1002/ecs2.2556
Narayan S., Beck M. W., Wilson P., Thomas C. J., Guerrero A., Shepard C. C., et al. (2017). The Value of Coastal Wetlands for Flood Damage Reduction in the Northeastern USA. Sci. Rep. 7, 9463. doi: 10.1038/s41598-017-09269-z
Nellemann C., Corcoran E., Duarte C. M., Valdrés L., Young C. D., Fonseca L., et al. (2009). Blue Carbon: The Role of Healthy Oceans in Binding Carbon Bergen: UN Environment, GRID-Arendal). Available at: https://portals.iucn.org/library/sites/library/files/documents/2009-052.pdf.
Nielsen R., Kristiansen A., Mathiesen L., Mathiesen H. (1995). Distributional Index of the Benthic Macroalgae of the Baltic Sea Area. Acta Bot. Fenn. 155, 1–51.
Norderhaug K. M., Nedreaas K., Huserbråten M., Moland E. (2021). Depletion of Coastal Predatory Fish Sub-Stocks Coincided With the Largest Sea Urchin Grazing Event Observed in the NE Atlantic. Ambio 50, 163–173. doi: 10.1007/s13280-020-01362-4
Norderhaug K. M., van Son T. C., Nikolioudakis N., Thormar J., Moy F., Knutsen J. A., et al. (2020). Biomassemodell for Stortare - Ressursmodell for Fremtidens Forvaltning. Rapport Fra Havforskningen 2020-7 (Norway: Institute of Marine Research). Available at: https://hdl.handle.net/11250/2685899.
Nordlund L. M., Koch E. W., Barbier E. B., Creed J. C. (2016). Seagrass Ecosystem Services and Their Variability Across Genera and Geographical Regions. PLoS One 11, e0163091. doi: 10.1371/journal.pone.0163091
Nyqvist A., André C., Gullstrom M., Baden S. P., Åberg P. (2009). Dynamics of Seagrass Meadows on the Swedish Skagerrak Coast. Ambio 38, 85–88. doi: 10.1579/0044-7447-38.2.85
Öberg J. (2006). Primary Production by Macroalgae in Kattegat, Estimated From Monitoring Data, Seafloor Properties, and Model Simulations. Cont. Shelf Res. 26, 2415–2432. doi: 10.1016/j.csr.2006.07.005
Ørberg S. B., Krause-Jensen D., Geraldi N. R., Ortega A., Díaz-Rúa R., Duarte C. M. (2022). Fingerprinting Arctic and North Atlantic Macroalgae with eDNA–Application and perspectives. Environmental DNA 4 (2), 385–401. doi: 10.1002/edn3.262
Olafsen T., Winther U., Olsen Y., Skjermo J. (2012) Verdiskaping Basert På Produktive Hav I 2050. Rapport Fra Arbeidsgruppe Oppnevnt Av Det Kongelige Norske Videnskabers Selskap (DKNVS) Og Norges Tekniske Vitenskapsakademi (NTVA). Available at: www.sintef.no/globalassets/upload/fiskeri_og_havbruk/publikasjoner/verdiskaping-basert-pa-produktive-hav-i-2050.pdf.
Olesen B., Krause-Jensen D., Marbà N., Christensen P. B. (2015). Eelgrass Zostera Marina in Subarctic Greenland: Dense Meadows With Slow Biomass Turnover in Cold Waters. Mar. Ecol. Prog. Ser. 518, 107–121. doi: 10.3354/meps11087
Olsen J. L., Stam W. T., Coyer J. A., Reusch T. B. H., Billingham M., Boström C., et al. (2004). North Atlantic Phylogeography and Large-Scale Population Differentiation of the Seagrass Zostera Marina L. Mol. Ecol. 13, 1923–1941. doi: 10.1111/j.1365-294X.2004.02205.x
Oreska M. P. J., McGlathery K. J., Aoki L. R., Berger A. C., Berg P., Mullins L. (2020). The Greenhouse Gas Offset Potential From Seagrass Restoration. Sci. Rep. 10, 7325. doi: 10.1038/s41598-020-64094-1
Ortega A., Geraldi N. R., Duarte C. M. (2020). Environmental DNA Identifies Marine Macrophyte Contributions to Blue Carbon Sediments. Limnol. Oceanogr. 65 (12), 3139–3149. doi: 10.1002/lno.11579
Orth R. J., Carruthers T. J. B., Dennison W. C., Duarte C. M., Fourqurean J. W., Heck K. L., et al. (2006). A Global Crisis for Seagrass Ecosystems. Bioscience 56, 987–996. doi: 10.1641/0006-3568(2006)56[987:Agcfse]2.0.Co;2
Orth R. J., Lefcheck J. S., McGlathery K. S., Aoki L., Luckenbach M. W., Moore K. A., et al. (2020). Restoration of Seagrass Habitat Leads to Rapid Recovery of Coastal Ecosystem Services. Sci. Adv. 6, eabc6434. doi: 10.1126/sciadv.abc6434
Ostenfeld C. H. (1908). The Land-Vegetation of the Færöes: With Special Reference to the Higher Plants. Bot. Færöes 3, 867–1026.
Ottósson J. G., Sveinsdóttir A., Harðardóttir M. (Eds.) (2016). Vistgerðir Á Íslandi. Fjölrit Náttúrufræðistofnunar Nr. 54 (Náttúrufræðistofnun Íslands: Garðabær). Available at: http://utgafa.ni.is/fjolrit/Fjolrit_54.pdf.
Paine E. R., Schmid M., Boyd P. W., Diaz-Pulido G., Hurd C. L. (2021). Rate and Fate of Dissolved Organic Carbon Release by Seaweeds: A Missing Link in the Coastal Ocean Carbon Cycle. J. Phycol. 57, 1375–1391. doi: 10.1111/jpy.13198
Pätsch R., Schaminée J. H. J., Janssen J. A. M., Hennekens S. M., Bruchmann I., Jutila H., et al. (2019). Between Land and Sea ? A Classification of Saline and Brackish Grasslands of the Baltic Sea Coast. Phytocoenologia 49, 319–348. doi: 10.1127/phyto/2019/0339
Pedersen M. F., Filbee-Dexter K., Norderhaug K. M., Fredriksen S., Frisk N. L., Fagerli C. W., et al. (2020). Detrital Carbon Production and Export in High Latitude Kelp Forests. Oecologia 192, 227–239. doi: 10.1007/s00442-019-04573-z
Perry D., Hammar L., Linderholm H. W., Gullström M. (2020). Spatial Risk Assessment of Global Change Impacts on Swedish Seagrass Ecosystems. PLoS One 15, e0225318. doi: 10.1371/journal.pone.0225318
Perry D., Staveley T., Deyanova D., Baden S., Dupont S., Hernroth B., et al. (2019). Global Environmental Changes Negatively Impact Temperate Seagrass Ecosystems. Ecosphere 10, e02986. doi: 10.1002/ecs2.2986
Petersen C. G. J. (1914). “Om Bændeltangens (Zostera Marina) Aarsproduktion I De Danske Farvande. Kap. Ix,” in Mindeskrift I Anledning Af Hundredaaret for Japetus Stenstrups Fødsel. Eds. Jungersen H., Warming E. (København: Bianco Lunos Bogtrykkeri), 1–20.
Queirós A. M., Stephens N., Widdicombe S., Tait K., McCoy S. J., Ingels J., et al (2019). Connected Macroalgal-Sediment Systems: Blue Carbon and Food Webs in the Deep Coastal Ocean. Ecol. Monogr. 89 (3), p.e01366. doi: 10.1002/ecm.1366
Reusch T. B. H., Bostrôm C. (2011). Widespread Genetic Mosaicism in the Marine Angiosperm Zostera Marina is Correlated With Clonal Reproduction. Evol. Ecol. 25, 899–913. doi: 10.1007/s10682-010-9436-8
Reusch T. B. H., Boström C., Stam W. T., Olsen J. L. (1999). An Ancient Eelgrass Clone in the Baltic. Mar. Ecol. Prog. Ser. 183, 301–304. doi: 10.3354/meps183301
Reusch T. B. H., Dierking J., Andersson H. C., Bonsdorff E., Carstensen J., Casini M., et al. (2018). The Baltic Sea as a Time Machine for the Future Coastal Ocean. Sci. Adv. 4, eaar8195. doi: 10.1126/sciadv.aar8195
Rhein M., Rintoul S. R., Aoki S., Campos E., Chambers D., Feely R. A., et al. (2014). “Observations: Ocean,” in Climate Change 2013: The Physical Science Basis. Contribution of Working Group I to the Fifth Assessment Report of the Intergovernmental Panel on Climate Change. Eds. Stocker T. F., Qin D., Plattner G.-K., Tignor M., Allen S. K., Boschung J. (Cambridge, United Kingdom and New York, NY, USA: Cambridge University Press), 255–316. doi: 10.1017/CBO9781107415324.010
Riemann B., Abay A. T., Ankjærø T., Bruhn A., Dahl K., Galatius A., et al. (2020). Regional Havplanlægning I Det Vestlige Kattegat – Natur-, Erhvervs- Og Samfundsmæssige Forhold Og Scenarier (Aarhus Universitet, DCE – Nationalt Center for Miljø og Energi. Scientific Report no. 403). Available at: https://dce2.au.dk/pub/SR403.pdf.
Riemann B., Carstensen J., Dahl K., Fossing H., Hansen J. W., Jakobsen H. H., et al. (2016). Recovery of Danish Coastal Ecosystems After Reductions in Nutrient Loading: A Holistic Ecosystem Approach. Estuar Coast 39, 82–97. doi: 10.1007/s12237-015-9980-0
Rinde E., Christie H., Fagerli C. W., Bekkby T., Gundersen H., Norderhaug K. M., et al. (2014). The Influence of Physical Factors on Kelp and Sea Urchin Distribution in Previously and Still Grazed Areas in the NE Atlantic. PLoS One 9, e100222. doi: 10.1371/journal.pone.0100222
Rinne H., Salovius-Lauren S. (2020). The Status of Brown Macroalgae Fucus Spp. And its Relation to Environmental Variation in the Finnish Marine Area, Northern Baltic Sea. Ambio 49, 118–129. doi: 10.1007/s13280-019-01175-0
Röhr M. E., Boström C., Canal-Vergés P., Holmer M. (2016). Blue Carbon Stocks in Baltic Sea Eelgrass (Zostera Marina) Meadows. Biogeosciences 13, 6139–6153. doi: 10.5194/bg-13-6139-2016
Röhr M. E., Holmer M., Baum J. K., Björk M., Boyer K., Chin D., et al. (2018). Blue Carbon Storage Capacity of Temperate Eelgrass (Zostera Marina) Meadows. Global Biogeochem. Cycles 32, 1457–1475. doi: 10.1029/2018gb005941
Ruiz-Frau A., Gelcich S., Hendriks I. E., Duarte C. M., Marbà N. (2017). Current State of Seagrass Ecosystem Services: Research and Policy Integration. Ocean Coast. Manage. 149, 107–115. doi: 10.1016/j.ocecoaman.2017.10.004
Sahla M., Tolvanen H., Ruuskanen A., Kurvinen L. (2020). Assessing Long Term Change of Fucus Spp. Communities in the Northern Baltic Sea Using Monitoring Data and Spatial Modeling. Estuar Coast Shelf S. 245, 107023. doi: 10.1016/j.ecss.2020.107023
Salinas C., Duarte C. M., Lavery P. S., Masque P., Arias-Ortiz A., Leon J. X., et al. (2020). Seagrass Losses Since Mid-20th Century Fuelled CO2 Emissions From Soil Carbon Stocks. Glob. Change Biol. 26, 4772–4784. doi: 10.1111/gcb.15204
Sayre R., Noble S., Hamann S., Smith R., Wright D., Breyer S., et al. (2019). A New 30 Meter Resolution Global Shoreline Vector and Associated Global Islands Database for the Development of Standardized Ecological Coastal Units. J. Oper Oceanogr 12, S47–S56. doi: 10.1080/1755876x.2018.1529714
Schernewski G., Bartel C., Kobarg N., Karnauskaite D. (2018). Retrospective Assessment of a Managed Coastal Realignment and Lagoon Restoration Measure: The Geltinger Birk, Germany. J. Coast. Conserv 22, 157–167. doi: 10.1007/s11852-017-0496-6
Schramm W. (1998). “Seaweed Ressources of the Baltic Sea and the German Coasts of the North Sea,” in Seaweed Ressources of the World. Eds. Critchley A. T., Ohno H. (Japan International Collaboration Agency), 226–232.
Schulman A., Alanen A., Hæggström C.-A., Huhta A.-P., Jantunen J., Kekäläinen H., et al. (2008). “Perinnebiotoopit,” in Suomen Luontotyyppien Uhanalaisuus – Osa 2: Luontotyyppien Kuvaukset (Assessment of Threatened Habitat Types in Finland – Part 2: Habitat Type Descriptions), SYKE Report 2/2011. Eds. Raunio A., Schulman A., Kontula T. (Helsinki: Suomen ympäristökeskus), 397–465.
Sejr M. K., Mouritsen K. N., Krause-Jensen D., Olesen B., Blicher M. E., Thyrring J. (2021). Small Scale Factors Modify Impacts of Temperature, Ice Scour and Waves and Drive Rocky Intertidal Community Structure in a Greenland Fjord. Front. Mar. Sci. 7. doi: 10.3389/fmars.2020.607135
Smale D. A., Burrows M. T., Moore P., O'Connor N., Hawkins S. J. (2013). Threats and Knowledge Gaps for Ecosystem Services Provided by Kelp Forests: A Northeast Atlantic Perspective. Ecol. Evol. 3, 4016–4038. doi: 10.1002/ece3.774
Staehr P. A., Göke C., Holbach A. M., Krause-Jensen D., Timmermann K., Upadhyay S., et al. (2019). Habitat Model of Eelgrass in Danish Coastal Waters: Development, Validation and Management Perspectives. Front. Mar. Sci. 6. doi: 10.3389/fmars.2019.00175
Steen H. (2018). Assessment of C-Fields for Kelp Harvesting in Sør-Trøndelag and Nord-Trøndelag in 2018. IMR Report Series (Bergen: Institute of Marine Research. Rapport fra Havforskningen no. 32-2018). Available at: www.hi.no/hi/nettrapporter/rapport-fra-havforskningen/2018/32-2018_rapportc-tarehostefelt_trondelag.
Steen H., Moy F. E., Bodvin T., Husa V. (2016). Regrowth After Kelp Harvesting in Nord-Trondelag, Norway. ICES J. Mar. Sci. 73, 2708–2720. doi: 10.1093/icesjms/fsw130
Steen H., Norderhaug K. M., Moy F. E. (2018). Tareundersøkelser I Nordland I 2018. Institute of Marine Research. Rapport Fra Havforskningen No. 44-2018.
Stewart-Sinclair P. J., Purandare J., Bayraktarov E., Waltham N., Reeves S., Statton J., et al. (2020). Blue Restoration - Building Confidence and Overcoming Barriers. Front. Mar. Sci. 7. doi: 10.3389/fmars.2020.541700
The BACC II Author Team (2015). Second Assessment of Climate Change for the Baltic Sea Basin (Cham: Springer International Publishing). doi: 10.1007/978-3-319-16006-1
Thormar J., Hasler-Sheetal H., Baden S., Boström C., Clausen K. K., Krause-Jensen D., et al. (2016). Eelgrass (Zostera Marina) Food Web Structure in Different Environmental Settings. PLoS One 11, e0146479. doi: 10.1371/journal.pone.0146479
Thyrring J., Wegeberg S., Blicher M. E., Krause-Jensen D., Hogslund S., Olesen B., et al. (2021). Latitudinal Patterns in Intertidal Ecosystem Structure in West Greenland Suggest Resilience to Climate Change. Ecography 44, 1156–1168. doi: 10.1111/ecog.05381
Tobler W. (1987). Measuring spatial resolution. Proceedings, Land Resources Information Systems Conference, Beijing, pp. 12–16.
Torn K., Krause-Jensen D., Martin G. (2006). Present and Past Depth Distribution of Bladderwrack (Fucus Vesiculosus) in the Baltic Sea. Aquat. Bot. 84, 53–62. doi: 10.1016/j.aquabot.2005.07.011
UNESCO (2021). UNESCO Marine World Heritage: Custodians of the Globe’s Blue Carbon Assets (Paris, France: United Nations Educational, Scientific and Cultural Organization).
Valiela I., Lloret J., Bowyer T., Miner S., Remsen D. P., Elmstrom E., et al. (2018). Transient Coastal Landscapes: Rising Sea Level Threatens Salt Marshes. Sci. Total Environ., 640–641, 1148–1156. doi: 10.1016/j.scitotenv.2018.05.235
van der Heide T., Temmink R. J. M., Fivash G. S., Bouma T. J., Boström C., Didderen K., et al. (2021). Coastal Restoration Success via Emergent Trait-Mimicry is Context Dependent. Biol. Conserv. 264, 109373. doi: 10.1016/j.biocon.2021.109373
van Katwijk M. M., Thorhaug A., Marbà N., Orth R. J., Duarte C. M., Kendrick G. A., et al. (2016). Global Analysis of Seagrass Restoration: The Importance of Large-Scale Planting. J. Appl. Ecol. 53, 567–578. doi: 10.1111/1365-2664.12562
Verbeek J., Louro I., Christie H., Carlsson P. M., Matsson S., Renaud P. E. (2021). Restoring Norway’s Underwater Forests a Strategy to Recover Kelp Ecosystems From Urchin Barrens (Oslo: SeaForester, NIVA, Akvaplan-niva). Available at: www.niva.no/en/reports/restoring-norways-underwater-forests/_/attachment/download/bd43a73d-631d-46da-abde-ffe2e9774243:9ab75e57c149a6523927382b1fb17c272aaed95d/Restoring%20Norway's%20Underwater%20Forests_light.pdf.
Vestergaard P. (2000). Strandenge – En Beskyttet Naturtype. Miljø-Og Energiministeriet, Skov- Og Naturstyrelsen (Copenhagen, Denmark: G.E.C. Gads Forlag).
Vistgerdakort.ni.is (2018) Vistgerðakort. Available at: https://vistgerdakort.ni.is/.
Waern M. (1952). Rocky-Shore Algae in the Öregrund Archipelago. [Dissertation] (Uppsala, Sweden: Sv. växtgeografiska sällsk).
Walday M. G., Fagerli C. W., Frigstad H., Staalstrøm A., Kaurin M., Christensen G., et al. (2020). Evaluering Av ØKOKYST - Stasjonsnett Og Klassegrenser. NIVA Report No. 7547-2020 (Oslo: Norwegian Institute of Water Research). Available at: https://hdl.handle.net/11250/2683488.
Ward R. D. (2020). Carbon Sequestration and Storage in Norwegian Arctic Coastal Wetlands: Impacts of Climate Change. Sci. Total Environ. 748, 141343. doi: 10.1016/j.scitotenv.2020.141343
Watanuki A., Aota T., Otsuka E., Kawai T., Iwahashi Y., Kuwahara H., et al. (2010). Restoration of Kelp Beds on an Urchin Barren: Removal of Sea Urchins by Citizen Divers in Southwestern Hokkaido. Bull. Fish. Res. Agen. (Japan) 32, 83–87.
Water Framework Directive (2000). Directive 2000/60/EC of the European Parliament and the Council of 23 October 2000 Establishing a Framework for Community Action in the Field of Water Policy. Off. J. Eur. Communities L 327, 1–73. https://www.legislation.gov.uk/eudr/2000/60/contents
Waycott M., Duarte C. M., Carruthers T. J. B., Orth R. J., Dennison W. C., Olyarnik S., et al. (2009). Accelerating Loss of Seagrasses Across the Globe Threatens Coastal Ecosystems. Proc. Natl. Acad. Sci. 106, 12377–12381. doi: 10.1073/pnas.0905620106
Weinberger F., Paalme T., Wikström S. A. (2020). Seaweed Resources of the Baltic Sea, Kattegat and German and Danish North Sea Coasts. Bot. Mar. 63, 61–72. doi: 10.1515/bot-2019-0019
Wernberg T., Krumhansl K., Filbee-Dexter K., Pedersen M. F. (2019). “Status and Trends for the World’s Kelp Forests,” in World Seas: An Environmental Evaluation. Volume III: Ecological Issues and Environmental Impacts, 2. ed. Ed. Sheppard C. (Amsterdam: Elsevier), 57–78. doi: 10.1016/B978-0-12-805052-1.00003-6
Wickström S. A., Carstensen J., Blomqvist M., Krause-Jensen D. (2016). Cover of Coastal Vegetation as an Indicator of Eutrophication Along Environmental Gradients. Mar. Biol. 163, 257. doi: 10.1007/s00227-016-3032-6
Woodroffe S. A., Long A. J. (2009). Salt Marshes as Archives of Recent Relative Sea Level Change in West Greenland. Quat. Sci. Rev. 28, 1750–1761. doi: 10.1016/j.quascirev.2009.02.009
Young A. P., Carilli J. E. (2019). Global Distribution of Coastal Cliffs. Earth Surface Processes Landforms 44, 1309–1316. doi: 10.1002/esp.4574
Yu S. Y., Berglund B. E., Andren E., Sandgren P. (2004). Mid-Holocene Baltic Sea Transgression Along the Coast of Blekinge, SE Sweden Ancient Lagoons Correlated With Beach Ridges. GFF 126, 257–272. doi: 10.1080/11035890401263257
Keywords: eelgrass, salt marsh, macroalgae, area distribution, carbon stock, carbon sequestration, ecosystem services, management
Citation: Krause-Jensen D, Gundersen H, Björk M, Gullström M, Dahl M, Asplund ME, Boström C, Holmer M, Banta GT, Graversen AEL, Pedersen MF, Bekkby T, Frigstad H, Skjellum SF, Thormar J, Gyldenkærne S, Howard J, Pidgeon E, Ragnarsdóttir SB, Mols-Mortensen A and Hancke K (2022) Nordic Blue Carbon Ecosystems: Status and Outlook. Front. Mar. Sci. 9:847544. doi: 10.3389/fmars.2022.847544
Received: 02 January 2022; Accepted: 20 April 2022;
Published: 31 May 2022.
Edited by:
William Richard Turrell, Marine Scotland, United KingdomReviewed by:
Mat Vanderklift, Commonwealth Scientific and Industrial Research Organisation (CSIRO), AustraliaAlejandro H. Buschmann, University of Los Lagos, Chile
W. Judson Kenworthy, Independent researcher, Beaufort, NC, United States
Copyright © 2022 Krause-Jensen, Gundersen, Björk, Gullström, Dahl, Asplund, Boström, Holmer, Banta, Graversen, Pedersen, Bekkby, Frigstad, Skjellum, Thormar, Gyldenkærne, Howard, Pidgeon, Ragnarsdóttir, Mols-Mortensen and Hancke. This is an open-access article distributed under the terms of the Creative Commons Attribution License (CC BY). The use, distribution or reproduction in other forums is permitted, provided the original author(s) and the copyright owner(s) are credited and that the original publication in this journal is cited, in accordance with accepted academic practice. No use, distribution or reproduction is permitted which does not comply with these terms.
*Correspondence: Dorte Krause-Jensen, dkj@ecos.au.dk